Introduction
All insects have detoxification abilities that vary among species and developmental stages (Yu & Hsu, Reference Yu and Hsu1993; Casida & Quistad, Reference Casida and Quistad2004). The ability to degrade toxic substances is essential to the survival of insect pests in the constantly changing chemical environment of the agroecosystem. Metabolism of toxic compounds involves two phases. Phase I is the addition of a polar group to the substrate or its cleavage. Phase II is the addition of sugar, amino acid, sulfate, or phosphate groups to the products resulting from phase I, to increase hydrophilicity so as to facilitate excretion by the insect (Bernard & Philogène, Reference Bernard and Philogène1993). The three most important detoxification enzyme families in insects are polysubstrate monooxygenases (PSMO) [also called cytochrome P450 monooxygenases (P450)], carboxylesterases (EST), and glutathione-S-transferases (GST) (Després et al., Reference Després, David and Gallet2007). PSMO and EST are involved in phase I, whereas GST is involved in phase II (Bernard & Philogène, Reference Bernard and Philogène1993). These enzyme families are subject to gene amplification, or overexpression, and variations in the coding sequence that can modify their detoxification capabilities (Li et al., Reference Li, Schuler and Berenbaum2007).
Metabolic enzymes changes have emphasized the importance of pest-detoxification capabilities in the development of pesticide-resistant populations (Scott, Reference Scott1999; Enayati et al., Reference Enayati, Ranson and Hemingway2005; Montella et al., Reference Montella, Schama and Valle2012). Several in vivo methods using enzyme inhibitors to gauge insecticide susceptibility and biochemical in vitro methods to detect metabolic-detoxification enzyme changes are available to examine pesticide resistance (Brown & Brogdon, Reference Brown and Brogdon1987). Several studies report that metabolic enzymes are involved in the detoxification of insecticides with different modes of action (MoA) in various lepidopteran pests (Biddinger et al., Reference Biddinger, Hull and McPheron1996; Ahmad & Hollingworth, Reference Ahmad and Hollingworth2004; Reyes et al., Reference Reyes, Franck, Charmillot, Ioriatti, Olivares, Pasqualini and Sauphanor2007; Siegwart et al., Reference Siegwart, Monteiro, Maugin, Olivares, Malfitano-Carvalho and Sauphanor2011), and in various insect developmental stages (Karoly et al., Reference Karoly, Rose, Thompson, Hodgson, Rock and Roe1996; Yu et al., Reference Yu, Nguyen and Abo-Elghar2003; Rodríguez et al., Reference Rodríguez, Bosch, Sauphanor and Avilla2010). Metabolic enzymes also help to elucidate the metabolic status of susceptible pest species (Usmani & Knowles, Reference Usmani and Knowles2001; Guo et al., Reference Guo, Chai, Zhang, Zhao, Gao and Ma2017; Vojoudi et al., Reference Vojoudi, Saber, Gharekhani and Esfandiari2017). Furthermore, the enzymes can be used to discover which metabolic groups are involved in resistance, comparing metabolic status among susceptible and resistant species (Sauphanor et al., Reference Sauphanor, Cuany, Bouvier, Brosse, Amichot and Bergé1997; Ahmad & Hollingworth, Reference Ahmad and Hollingworth2004; Reuveny & Cohen, Reference Reuveny and Cohen2004).
Chemicals that inhibit specific detoxification pathways are important in researching detoxification mechanisms, resistance, and MoA in insecticide studies (Metcalf, Reference Metcalf1967; Snoeck et al., Reference Snoeck, Greenhalgh, Tirry, Clark, Van Leeuwen and Dermauw2017). Chemical inhibition is particularly useful in this context due to the diverse range of chemical structures and metabolic routes of insecticides (Roberts & Huston, Reference Roberts and Hutson1998). The most common inhibitors (also called synergists) for EST, GST, and PSMO enzymes are S,S,S-tributyl phosphorotrithioate (DEF), diethyl maleate (DEM), and piperonyl butoxide (PBO), respectively (Bernard & Philogène, Reference Bernard and Philogène1993; Wu et al., Reference Wu, Miyata, Kang and Xie2007; Sial & Brunner, Reference Sial and Brunner2012; Snoeck et al., Reference Snoeck, Greenhalgh, Tirry, Clark, Van Leeuwen and Dermauw2017).
Cydia pomonella (L.), Grapholita molesta (Busck), and Lobesia botrana (Denis & Schiffermüller) are three key tortricid moth pest species which target Mediterranean fruit crops. They adversely affect mainly apples, peaches, and grapes, respectively, but have worldwide distribution hosts (Ioriatti et al., Reference Ioriatti, Anfora, Tasin, de Cristofaro, Witzgall and Lucchi2011; Kirk et al., Reference Kirk, Dorn and Mazzi2013; Damos et al., Reference Damos, Colomar and Ioriatti2015). Toxin susceptibility and metabolic enzyme activity commonly vary in different insect species (Yu, Reference Yu2008). The specific enzymatic activity of an individual may be linked to its diet (Krieger et al., Reference Krieger, Feeny and Wilkinson1971; Rose, Reference Rose1985); besides, the use of insecticides can select for different enzymatic detoxification mechanisms (Xue et al., Reference Xue, Pang, Li and Liu2010). Therefore, to study the basal levels of enzymatic detoxification activities in different species, susceptible strains reared for a long time in the laboratory, fed with the same artificial diet, can be very useful. In contrast, the use of field populations may imply the co-occurrence of different resistance mechanisms, complicating the interpretation of results (Soderlund & Bloomquist, Reference Soderlund, Bloomquist, Roush and Tabashnik1990; Bosch et al., Reference Bosch, Avilla, Musleh and Rodríguez2018).
Navarro-Roldán et al. (Reference Navarro-Roldán, Avilla, Bosch, Valls and Gemeno2017) found significant differences in adult toxicity levels among these species for three neurotoxic insecticides that have different MoAs [chlorpyrifos (organophosphate, acetyl cholinesterase inhibitor), λ-cyhalothrin (pyrethroid, sodium channel modulator), and thiacloprid (neonicotinoid, nicotinic acetyl cholinesterase receptor agonist)]. These three insecticides are usually recommended for the control of two of the three moth species (MAPAMA, 2017). In addition, females of all three species were less susceptible than males to thiacloprid (maximum 3.4-fold), whereas, they were more susceptible than males to chlorpyrifos (maximum 41.5-fold) (Navarro-Roldán et al., Reference Navarro-Roldán, Avilla, Bosch, Valls and Gemeno2017). Higher female susceptibility to organophosphates had been reported previously in G. molesta (de Lame et al., Reference de Lame, Hong, Shearer and Brattsten2001). Comparative research of the use of enzyme inhibitors and metabolic detoxification is necessary, therefore, to present a more complete picture of the detoxification abilities of these three species. Although adults are not the primary target of these insecticides, they are exposed to them in the field, and the adult stage facilitates the evaluation of differences in sex response.
The main objective of our research was to study the metabolic mechanisms involved in susceptible strains of C. pomonella, G. molesta, and L. botrana regarding their defense against different neurotoxic insecticides (without any enzymatic induction by insecticides). These insect species have previously presented dissimilar levels of susceptibility to identical insecticides. Two approaches were followed. Firstly, we examined whether the enzymatic inhibition using DEF, DEM, and PBO affected insecticide-induced mortality. Secondly, we quantified EST, GST, and PSMO baseline activity in untreated individuals and in those treated with enzyme inhibitors.
Materials and methods
Insects
Susceptible laboratory strains of C. pomonella, G. molesta, and L. botrana collected in Lleida (Spain), Piacenza (Italy), and La Rioja (Spain), respectively, were maintained under laboratory conditions for more than 5 years, without the introduction of wild individuals. Larvae were reared on an artificial diet (Ivaldi-Sender, Reference Ivaldi-Sender1974) in a growth chamber. Insects used in Lleida (mortality bioassays) were maintained at 25 ± 1°C. Those used in Avignon (quantification of enzymatic activity) were shipped from Lleida as larvae or pupae and were maintained at 27.5 ± 0.5°C, under a 16:8 h light:dark photoperiod. Pupae were separated by sex and checked daily for adult emergence, except for C. pomonella, which was separated at the adult stage, also on a daily basis. Adults were treated zero to 24 h post-emergence, during the first half of the photophase.
Insecticides and enzyme inhibitors
As insecticide active ingredients we used chlorpyrifos [TraceCERT® certified reference material, ≈100% (a.i.)], λ-cyhalothrin [PESTANAL® analytical standard, ≈100% (a.i.)], and thiacloprid [PESTANAL® analytical standard, ≈100% (a.i.)]. The enzyme-inhibitors were DEF [analytical standard, 97% (a.i.)], DEM [analytical standard, 97% (a.i.)], and PBO [technical grade, 90% (a.i.)] all of them distributed by Sigma-Aldrich, Madrid, Spain. All dilutions used in the bioassays were prepared from pure compounds using acetone as a solvent (CHROMASOLV® for HPLC, ≥99.9%, Sigma-Aldrich). Dilutions were stored in 2 or 4 ml acetone-rinsed glass vials at 7°C. The same stock of acetone used to prepare the dilutions was also used as the negative control.
Effects of enzyme inhibitors on insecticide-induced insect mortality
Insecticides were applied at the LC50 (Navarro-Roldán et al., Reference Navarro-Roldán, Avilla, Bosch, Valls and Gemeno2017), and the inhibitors were applied at the highest concentration that produced the same mortality as the solvent did (table 1) (the concentration was determined in preliminary tests). The treatments containing one insecticide mixed with one inhibitor used the same concentration as when they were applied alone. Between 60 and 115 individuals per treatment were used for the 96 treatment groups [solvent, enzyme-inhibitor, insecticide (LC50), insecticide (LC50)+enzyme-inhibitor, species, and sex]. Different treatments were tested each day depending on insect availability. Tests were performed in groups of at least 3–10 insects of the same treatment set, with repetitions for each treatment set varying from 6 to 13, until the desired sample size was achieved.
Table 1. Concentration of enzyme inhibitor and insecticide used.

1 Highest concentration of inhibitor which did not produce significantly larger mortalities than solvent (N = 30, Fisher exact test). A range of 3–15 concentrations per inhibitor, species and sex, were used.
2 Insecticide LC50 (Navarro-Roldán, et al., Reference Navarro-Roldán, Avilla, Bosch, Valls and Gemeno2017).
One or two adults were placed in a 10 ml test tube, anesthetized with a brief (10 s) flow of industrial grade CO2 and placed upside down under a stereoscope. A 1 µl test solution was applied to the ventral thoracic region of each insect with a high-precision, positive displacement, repeatable-dispensing micropipette (Multipette® M4, Eppendorf, Germany). They were then transferred to a 150 ml polypropylene, non-sterile, clinical sample bottle (diameter, 57 mm; height, 73 mm). Three to ten individuals subjected to the same treatment were placed together in the same bottle. The lid of the bottle was punctured with ten holes (1 mm diameter) to allow gas exchange, and a 1.5 ml Eppendorf plastic vial, containing a 10% sugar solution and a cotton plug was placed on the bottom to supply nutrients during the observation period in the growth chamber.
Mortality was recorded 24 h after treatment. Adults observed with the naked eye were recorded as alive if they flew or walked apparently unaffected, or as moribund if they could barely walk or were lying on the bottom of the bottle but still moving. They were considered to be dead if they lay immobile on the bottom of the bottle. Mortality was estimated by adding together the number of moribund and dead insects.
Activity of detoxification enzyme families
Enzymatic activity was quantified by measuring the quantity of artificial substrate that converted to the product during the reaction time relative to the protein content of the sample extract. Whole abdomens of G. molesta and L. botrana were used, but in C. pomonella the anterior half of abdomen was used for the quantification of EST and GST activities, and the posterior half for PSMO. Abdomens were homogenized in the reaction solution (see below) in 1.5 ml Eppendorf tubes kept on ice. PSMO analysis was performed on the same day on fresh tissue, whereas GST and EST samples were frozen (−80°C) and analyzed once all samples were collected, to reduce sampling error (Reyes, Reference Reyes2007). The amount of product was determined by absorbance (EST and GST) and fluorescence (PSMO) using a microplate reader (Infinite 200, Tecan, Männedorf, Switzerland). After enzyme quantification, the total protein content of each sample was measured using the Bradford colorimetric absorbance test, using bovine serum albumin to build the standard curve (Bradford, Reference Bradford1976). Before protein quantification, the samples were diluted to place them within the range of standard curves. L. botrana male samples were undiluted, C. pomonella female samples were diluted tenfold, and the rest were diluted fivefold.
Carboxylesterases
Abdomens were homogenized in 110 µl of Hepes buffer (50 mM, pH 7.0) and centrifuged at 10,000 g for 15 min at 4°C. The supernatants were stored before use at −80°C. To measure total non-specific EST activity, 1 µl of supernatant, or 1 µl of Hepes buffer (50 mM, pH 7.0) for blanks (three wells), were placed in a transparent micro-plate (96-wells, Sterilin®, Newport, UK) containing 194 µl of α-naphthyl acetate (α-NA) 30 µM substrate (Ulrich & Weber, Reference Ullrich and Weber1972) in Hepes buffer (50 mM, pH 7.0). After a 20 min incubation period at 30°C in darkness, the reaction was stopped and the resulting solution colored by adding 55 µl of 0.2% Fast Garnet GBC, diluted in 2.5% sodium dodecyl sulfate. Absorbance of the reaction product (α-Naphtol) was measured at 590 nm. EST activity was expressed as nmol α-Naphtol min mg−1 of total protein using a standard curve of α-Naphtol (0–18 nmoles well−1). Between 55 and 59 insects per species and sex were used.
Glutathione-S-transferase
The extracts were prepared as for EST and 2 µl of supernatant, or 2 µl of Hepes buffer (50 mM, pH 7.0) for blanks (three wells), were placed in a transparent micro-plate (96-wells, Sterilin®, Newport, UK) containing 198 µl of 4-dinitro-chlorobenzene (CDNB) 0.76 mM substrate (Nauen & Stumpf, Reference Nauen and Stumpf2002) plus 2.52 mM glutathione (GSH) in Hepes buffer (50 mM, pH 7). After a 2 min incubation period at 25°C the absorbance of the reaction product (CDNB-glutathione (GSH) conjugate) was measured at 340 nm in the kinetic mode (every 30 s for 3 min). Since the CDNB-glutathione conjugate was not commercially available we were unable to build a standard curve, so we used the molar extinction coefficient (9.6 mM−1 × cm−1) of CDNB-glutathione to convert absorbance of CDNB-glutathione in μmol. The final specific activity was expressed in μmol of CDNB-glutathione min mg−1 of total protein extracted. Between 55 and 60 insects per species and sex were used.
Polysubstrate monooxygenases
Abdomens were homogenized in an incubation solution containing 7-ethoxycoumarin O-deethylation (0.4 mM) substrate (Bouvier et al., Reference Bouvier, Boivin, Beslay and Sauphanor2002) in Hepes buffer (50 mM, pH 7.0). Three blanks containing 100 µl of incubation solution were used. After a 4 h incubation period at 30°C the reaction was stopped with 100 µl of glycine buffer (1.5 M, pH 10.3) and centrifuged at 10,000 g for 5 min at room temperature. The supernatant (approximately 200 µl) was placed in black micro plates (96-wells, Corming Costar®, New York, USA). Fluorescence of the enzymatic product [7-hydroxycoumarin (7-HC)] was quantified with 380 nm excitation and 465 nm emission filters. PSMO activity was expressed as pg of 7-HC min mg−1 of total protein by using a standard curve of 7-HC (0.5–4.5 nmoles well−1). Between 57 and 60 insects per species and sex were used.
Effects of enzyme inhibitors on the activity of detoxification enzymes
To determine the effect of the enzyme inhibitors on enzymatic activity, adults were treated with inhibitors and after incubation, the enzymatic activities were measured as in the previous tests. The incubation period was 24 h for PSMO, EST, and GST (17–40 DEF and DEM-treated individuals). As PSMO-activity was not inhibited after 24 h (preliminary test, data not shown), a 1 h incubation period was assayed for PSMO (16–25 PBO-treated individuals). Time has been shown to play a role in enzymatic inhibition (Young et al., Reference Young, Gunning and Moores2005; Reference Young, Gunning and Moores2006; Bingham et al., Reference Bingham, Gunning, Delogu, Borzatta, Field and Moores2008), therefore an additional test was performed to explore the effect of time after inhibitor application on PSMO activity in both G. molesta and L. botrana (C. pomonella was not available at the time of this test). The time intervals between inhibitor application and enzyme activity quantification (i.e., incubation) for this test were 0, 0.5, 1, 2, 4, 12, and 16 h, along with an acetone control at 1 h (10–35 individuals per treatment).
Data analysis
All the statistical analyses were performed using R software (R Core Team, 2016). We used generalized linear models with Gaussian family functions for continuous variables (enzymatic activities), or binomial family functions for binomial variables (mortality percentage). The glht() and/or the predict means() functions performed Tukey's multiple pairwise comparisons, using multcomp and predictmeans R-packages, respectively for specified functions. Raw data and R scripts are available online (http://hdl.handle.net/10459.1/60223). Whenever the term ‘significant’ is used in the text regarding differences among treatments, it indicates a P-value <0.05. Correlations among mortality caused by enzyme-inhibitors and insecticide LD50 were made.
Results
Effects of enzyme inhibitors on insect mortality caused by insecticides
Table 2 shows the effect of the enzyme inhibitors on insect mortality, caused by insecticides. As it was expected, the inhibitors alone did not significantly increase mortality compared with solvent alone. This confirmed that the inhibitor concentrations used were not toxic in themselves. DEF, the EST inhibitor, significantly increased insecticide mortality in all cases, except in L. botrana females and C. pomonella males treated with thiacloprid (P-value = 0.876 and 0.773, respectively). DEM, the GST inhibitor, significantly synergized the activity of the three insecticides in G. molesta (except in females treated with λ-cyhalothrin). PBO, the PSMO inhibitor, had the same effect on males and females for all three species: it significantly synergized with thiacloprid, decreased the effect of chlorpyrifos, and did not affect λ-cyhalothrin (except in G. molesta females and L. botrana males).
Table 2. Mortality (%) of adult males and females of C. pomonella, G. molesta, and L. botrana 24 h after treatment with solvent acetone or insecticide (chlorpyrifos, λ-cyhalothrin, or thiacloprid), with and without detoxification enzyme inhibitors (DEF, DEM, and PBO).

P-values indicate the difference between the treatment with enzyme inhibitor (columns 2, 4, and 6) and the treatments without enzyme inhibitor (column 1). (N = 60–115 individuals/treatment).
Of the nine correlations made for the combination sex-species, comparing insecticide LD50 with enzyme inhibition dose, only thiacloprid and PSMO activity showed a high coefficient of determination (R 2 = 0.8989).
Activity of detoxification enzyme families
EST, GST, and PSMO activities in abdominal extracts of susceptible male and female adults of C. pomonella, G. molesta, and L. botrana were shown in fig. 1. In general, L. botrana was the species that had higher enzymatic activity. If we compare the enzymatic activity among species and sexes, L. botrana females had, in general, higher enzymatic activity than the rest of the groups, being these differences significant only for PSMO. The only observed differences between sexes were higher GST and PSMO levels in L. botrana females. EST activity was significantly higher in C. pomonella than in G. molesta, whereas the opposite was true for GST activity. PSMO activity was significantly higher in L. botrana females than in any other group, up to 5.3 times higher than in C. pomonella females. In addition, PSMO activity in L. botrana and G. molesta males was significantly higher than in C. pomonella males.
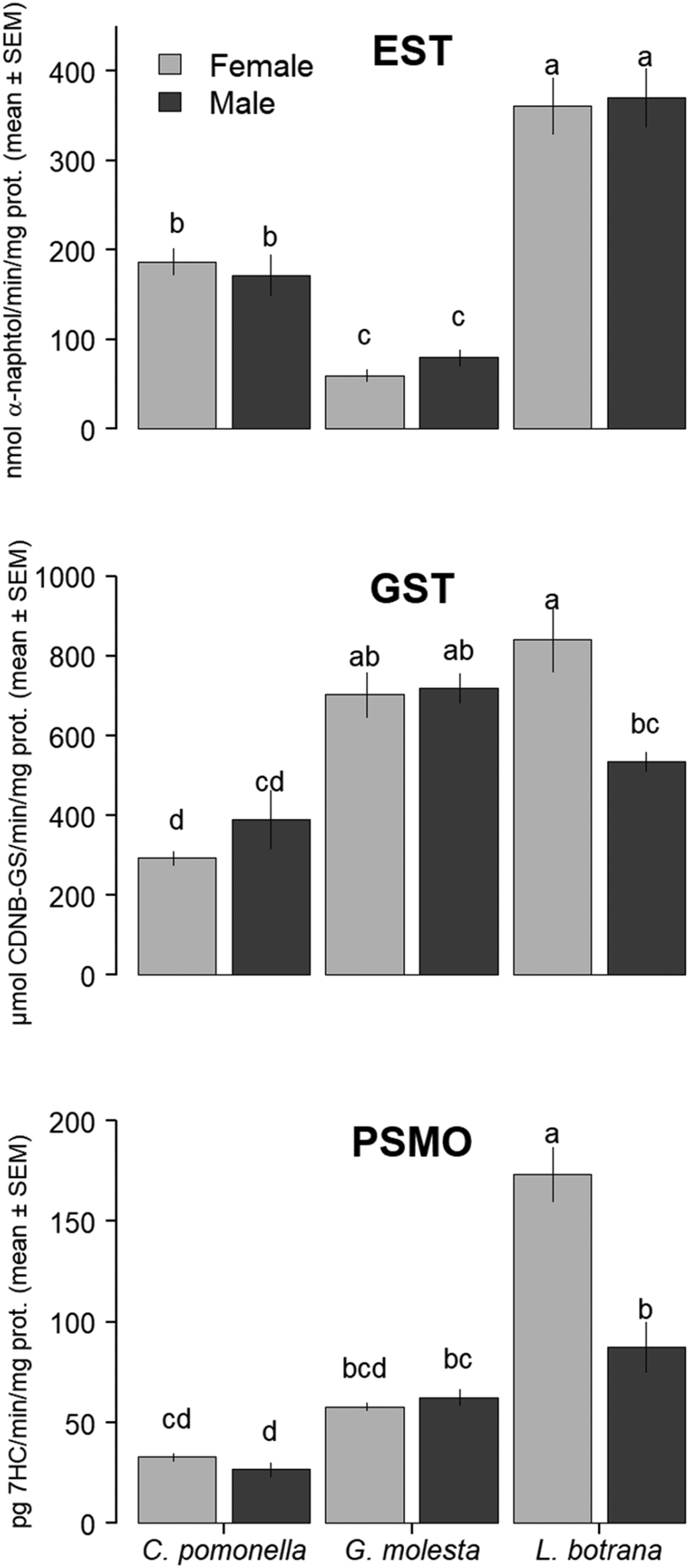
Fig. 1. EST, GST, and PSMO enzymatic activity in the abdomens of adult C. pomonella, G. molesta, and L. botrana from susceptible laboratory strains. Different letters indicate significant differences among bars for each enzyme family.
Effects of enzyme inhibitors on the activity of detoxification enzymes
After the 24 h incubation period, DEF significantly inhibited EST activity in both sexes for all species, obtaining enzymatic activity inhibition ratios ranging between 2.78 (G. molesta female) and 15.75 (L. botrana males). Inhibition in males was higher. Unexpectedly, DEM increased GST activity in G. molesta males but did not produce any observable effects in both sexes of the other species. After the 1 h incubation period, PBO did not have any effect on PSMO activity (table 3). These results coincide with those obtained a posteriori in the study of the effect of time after inhibitor application on PSMO activity in both G. molesta and L. botrana (fig. 2). A significant reduction in PSMO activity was obtained only 4 h after the PBO treatment in G. molesta females compared with the 0 h treatment, but not after 1 h PBO treatment. In G. molesta males this activity was significantly reduced from 4 h onward (fig. 2). On the contrary, in L. botrana, PSMO activity increased significantly with time (i.e., induction). For both sexes, this effect started to change at 12 h after treatment but was higher for males acquiring a 9.5-fold increase in relation to females 16 h after treatment (fig. 2).
Table 3. EST, GST, and PSMO activities on adult abdomens of susceptible male and female C. pomonella, G. molesta, and L. botrana adults, after application of DEF (24 h), DEM (24 h), and PBO (1 h), respectively.

P-values indicate differences between inhibitor-treated and control for each group (N = 16–40 individuals/treatment).
1 (−) Control treatment (only with acetone), (+) Inhibitor treatment DEF, DEM, or PBO for EST, GST, and MFO, respectively.
2 Enzymatic activities expressed in: EST (nmol α-naphtol min mg−1 protein), GST (μmol of CDNB-GS min mg−1 protein), and PSMO (pg of 7-HC min mg−1 protein).
3 Ratio of the enzymatic activity inhibition.
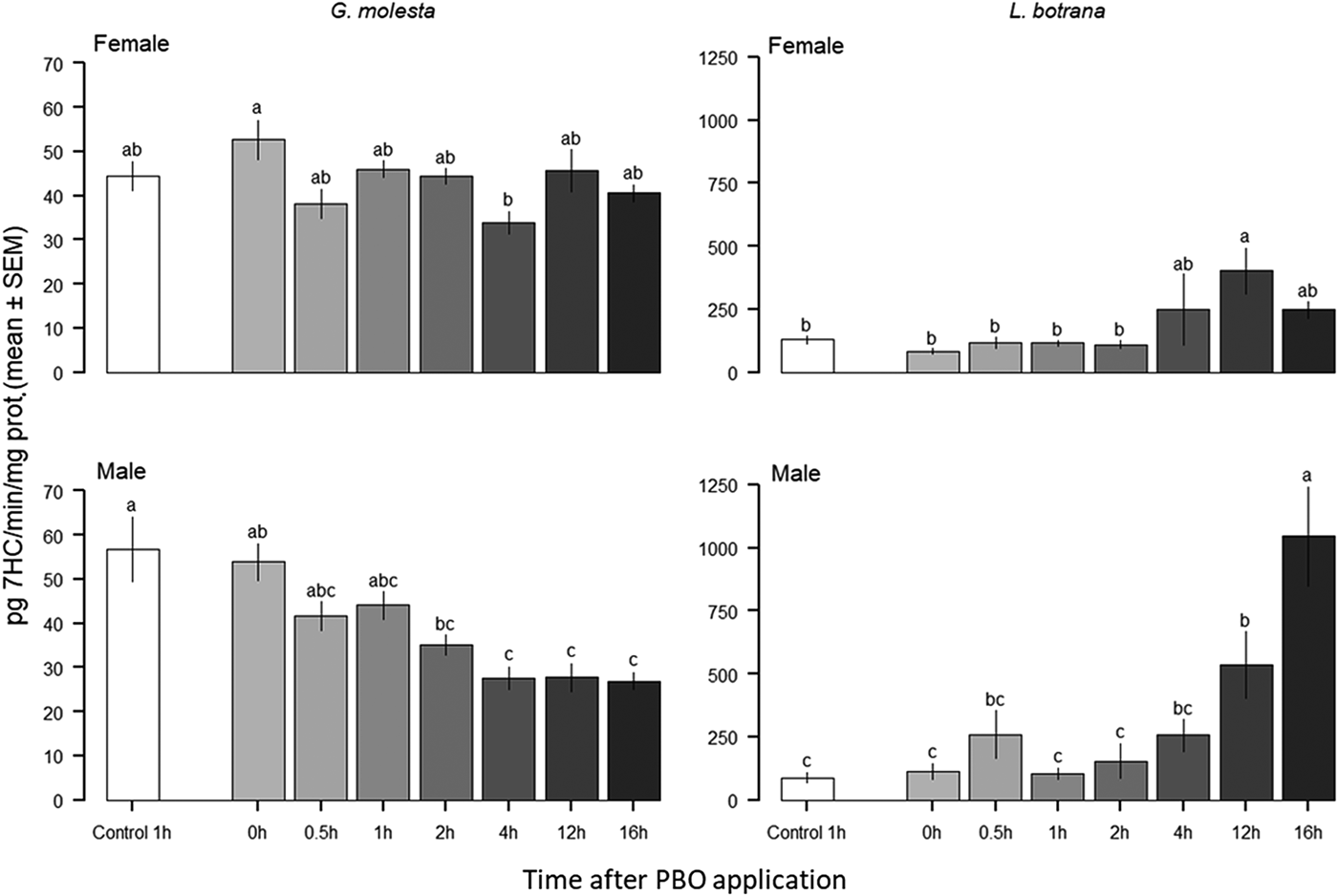
Fig. 2. Effects of time after application of PBO on PSMO enzymatic activity in the abdomens of adult G. molesta and L. botrana from susceptible laboratory strains. Different letters indicate significant differences among groups.
Discussion
The main aim of our study was to determine the metabolic mechanisms involved in insecticide detoxification in the adults of three susceptible tortricid moth pests that present varied susceptibility to the same insecticides, even at the sex level (Navarro-Roldán et al., Reference Navarro-Roldán, Avilla, Bosch, Valls and Gemeno2017). Comparing the baselines of the three species, L. botrana had the highest level of enzymatic activity, highlighting intense EST activity (fig. 1). L. botrana was the only species with enzymatic activity differences between sexes, with higher GST and PSMO activity in females. There are few published studies about insecticide resistance in L. botrana. Civolani et al. (Reference Civolani, Boselli, Butturini, Chicca, Fano and Cassanelli.2014) is the only case on resistance in field populations (http://www.pesticideresistance.com); and the present work along with Hatipoglu et al. (Reference Hatipoglu, Durmusoglu and Gürkan2015) are the only studies on the enzymatic activity of L. botrana. Nevertheless, in this case, no distinction was made between sexes to compare with our results. In addition, different units and a non-specified kind of microplate reader were used. G. molesta had lower EST activity than the other species but higher GST activity than in C. pomonella. In these two species, there were no differences between sexes in any measured enzymatic activity. On the contrary, all the codling moth populations analyzed in Reyes et al. (Reference Reyes, Barros-Parada, Ramírez and Fuentes-Contreras2015), one susceptible and six field populations, showed higher GST activity in females. De Lame et al. (Reference de Lame, Hong, Shearer and Brattsten2001) found higher EST activity in G. molesta males but any susceptible population was analyzed. The fact that most of the tested populations were collected from the field could be the reason why the results were not similar to ours. Siegward et al. (Reference Siegwart, Monteiro, Maugin, Olivares, Malfitano-Carvalho and Sauphanor2011) found higher PSMO activity in G. molesta females and Rodríguez et al. (Reference Rodríguez, Bosch, Sauphanor and Avilla2010) found no difference between the sexes in five field and one laboratory C. pomonella population. Nevertheless, in these two cases, the PSMO activity results were expressed per insect and not per unit weight of protein.
The significant reduction of EST activity when applying the synergist DEF, and the general increase in mortality of individuals simultaneously treated with DEF and insecticides, illustrate how EST plays a general role in detoxification by acting on all insecticides, for both sexes and all moth species examined. This non-specific action of the EST enzymatic family has been reported in other pest species. For example, EST is able to sequester several xenobiotic molecules in Myzus persicae (Sulzer), thus preventing contact of the insecticide with its molecular target, inducing resistance to a broad spectrum of insecticides (Devonshire & Moores, Reference Devonshire and Moores1982). The broad action of EST suggests that it is a cost-effective mechanism, easily adopted by several insect species. Gene amplification is an important mechanism for EST regulation in insects (Hemingway, Reference Hemingway2000). Its significance was demonstrated in Aedes aegypti L., in which up to 41 genes exhibiting gene amplification were linked to resistance to the pyrethroid deltamethrin (Faucon et al., Reference Faucon, Dusfour, Gaude., Navratil, Boyer, Chandre, Sirisopa, Thanispong, Juntarajumnong, Poupardin, Chareonviriyaphap, Girod, Corbel, Reynaud and David2015). However, one must remember that we used susceptible insect strains with a very basic enzymatic activity level; thus, enzyme activity may easily vary in populations subject to insecticide pressure.
Our mortality tests also showed how phase-I enzymatic activities (EST and PSMO) were involved in detoxification in all three species and in both sexes, whereas phase-II enzymes (GST) appeared to be less relevant in detoxification than the other two enzyme families, being important only in G. molesta. We reviewed 92 cases of detoxification mechanisms in Lepidoptera and found that GST was involved in only 36% of the cases; EST and PSMO were involved in a higher proportion, 63 and 64% of the cases, respectively (Navarro-Roldán, Reference Navarro-Roldán2017). It was interesting that in our enzymatic activity test with inhibitor-treated insects, the activity of GST in G. molesta males was enhanced with the application of DEM. The opposite was expected, given that in the mortality bioassays, with insecticide-plus-enzyme inhibitors, the application of DEM increased susceptibility to all the tested insecticides, and especially on the males. The increase in detoxification activity in response to environmental stressors such as plant compounds, insecticides and herbicides, corresponds to an increase in enzyme production (induction) that is responsible, at least in part, for host-plant selection and selective toxicity or resistance development to insecticides (Terriere, Reference Terriere1984; Yang et al., Reference Yang, Margolies, Zhu and Buschman2001; Yu, Reference Yu2004; Després et al., Reference Després, David and Gallet2007; Poupardin et al., Reference Poupardin, Reynaud, Strode, Ranson, Vontas and David2008). Synergists are compounds that enhance the toxicity of an insecticide but are nontoxic on their own (Matsumura, Reference Matsumura1985). However, these compounds do not always act as it is expected. For example, induction of enzymatic activities by the enzymatic inhibitor PBO was observed due to gene expression changes in Drosophila melanogaster (Meigen) (Willoughby et al., Reference Willoughby, Batterham and Daborn2007) and Bemisia tabaci (Zimmer et al., Reference Zimmer, Panini, Singh, Randall, Field, Roditakis, Mazzoni and Bass2017), but other synergist compounds have not yet been studied (Snoeck et al., Reference Snoeck, Greenhalgh, Tirry, Clark, Van Leeuwen and Dermauw2017). In addition, dose-dependent enzymatic activity induction (and inhibition) by insecticides has been observed in Plutella xylostella (L.) (Deng et al., Reference Deng, Zhang, Wu, Yu and Wu2016), and C. pomonella (Parra-Morales et al., Reference Parra-Morales, Alzogaray, Cichón, Garrido, Soleño and Montagna2017), both treated with the organophosphate chlorpyrifos.
Our results show that PBO produces a more diverse range of effects than the other two enzymatic synergists. PBO has a consistent and clear impact on the toxicity of thiacloprid and chlorpyrifos, resulting more variable with λ-cyhalothrin. In all species, for both sexes, the application of PBO leads to an increase in the toxicity of thiacloprid while the opposite occurs with chlorpyrifos. PBO is a well-known and widely used insecticide synergist, known to inhibit the activity of the insect cytochrome P450 detoxification system. PSMO reduction, caused by the action of the synergist, impacts insecticide efficacy. PSMO reduction decreases the activity of chlorpyrifos, due to a phenomenon known as bioactivation which has been described for organophosphates (Feyereisen, Reference Feyereisen1999) and detected in field populations with a high level of PSMO activity (Dunley & Welter, Reference Dunley and Welter2000; Bosch et al., Reference Bosch, Avilla, Musleh and Rodríguez2018). The complex chemical reactions of PSMO can lead to both insecticide bioactivation and detoxification (Levi et al., Reference Levi, Hollingworth and Hodgson1988), as it was seen in honeybees (Iwasa et al., Reference Iwasa, Motoyama, Ambrose and Roe2004). We found that any PSMO-activity change was detected 1 h after the application of PBO in all of the observed insect species (table 3). This was in spite of the change in mortality produced by both insecticides, chlorpyrifos, and thiacloprid (table 2). PBO has also been reported to act as an inhibitor of EST in Helicoverpa armigera (Hübner) and Bemisia tabaci (Gennadius) (Young et al., Reference Young, Gunning and Moores2005; Reference Young, Gunning and Moores2006). Hence, inhibition of EST by PBO can also occur and this should be examined in future work. Mortality was assessed 24 h after the application of the insecticide-plus-synergists and the enzymatic activity level measured 1 h after application of the synergist. Therefore, the effect of the inhibitor on the enzyme could have occurred at any time within this time interval. In a previous assay (data not shown), PBO treatment did not display a synergistic effect on PSMO enzymatic activity after 24 h. Due to this lack of inhibition, a test measuring the effect of time after PBO application on PSMO enzymatic activity was performed on G. molesta and L. botrana (fig. 2), the species available at the time of measurement. In G. molesta, we found a limited inhibition of 4 h after exposure. In L. botrana the PSMO activity increased for both sexes with a maximum of 9.5 times after 16 hours of PBO application for males. Hodgson & Levi (Reference Hodgson, Levi and Jones1998) found that, in mammals, PBO could function as a P450 inducer after a prolonged exposure to low doses, increasing the enzyme activity above the levels before the treatment. In addition, the PBO application is able to induce the expression of some detoxification gene families, as in the 32-fold one observed for the Cyp6A2 gene and for other PSMO genes by PBO in D. melanogaster (Willoughby et al., Reference Willoughby, Batterham and Daborn2007). These results could explain the lack of inhibitor effect in our first enzyme-inhibition experiment. The dependence of the efficacy of a synergist-insecticide upon a pre-treatment time was observed with PBO on pyrethroids in H. armigera (Young et al., Reference Young, Gunning and Moores2005; Reference Young, Gunning and Moores2006) and B. tabaci (Young et al., Reference Young, Gunning and Moores2006), PBO on carbamates in M. persicae and Aphis gossypii (Glover), and PBO on neonicotinoids in B. tabaci (Bingham et al., Reference Bingham, Gunning, Delogu, Borzatta, Field and Moores2008). Interestingly, the changes in enzymatic activity over time revealed sex differences: Inhibition could be detected 4 h after the treatment on G. molesta males, but not on females. Likewise, the different enzymatic activities between L. botrana males and females may help in illuminating sex differences in insecticide susceptibility, as it was reported previously (Navarro-Roldán et al., Reference Navarro-Roldán, Avilla, Bosch, Valls and Gemeno2017). Variations in enzymatic inhibition and induction observed in the kinetic experiment could help explain the differences in insecticide susceptibility between the sexes, as well as the lack of PMSO inhibition observed when enzyme quantification is made only once after inhibition. Nevertheless, further kinetic investigations involving the three species and the three enzyme inhibitors are needed to fully understand inhibitor-enzyme dynamics. As in other species, many ‘metabolic enzyme-inducers’ may be present and may influence the insect metabolic enzyme status in susceptible strains (Terriere, Reference Terriere1984; Yang et al., Reference Yang, Margolies, Zhu and Buschman2001; Yu, Reference Yu2004; Després et al., Reference Després, David and Gallet2007; Poupardin et al., Reference Poupardin, Reynaud, Strode, Ranson, Vontas and David2008; Xie et al., Reference Xie, Wang, Wu, Feng, Pan, Jiao, Zhou, Yang, Fu, Teng, Xu and Zhang2011; Deng et al., Reference Deng, Zhang, Wu, Yu and Wu2016; Parra-Morales et al., Reference Parra-Morales, Alzogaray, Cichón, Garrido, Soleño and Montagna2017).
In a previous study, Navarro-Roldán et al. (Reference Navarro-Roldán, Avilla, Bosch, Valls and Gemeno2017) reported significant differences in mortality (LD50) among insecticides (maximum 7800-fold), species (maximum 115-fold), and sexes (maximum 41.5-fold). In order to determine whether there is an association between enzymatic activity levels and mortality, we calculated the correlation between the LD50s of the three species and the two sexes for each enzyme family and insecticide (Navarro-Roldán et al., Reference Navarro-Roldán, Avilla, Bosch, Valls and Gemeno2017), along with the enzymatic activity reported herein. Of the nine regressions, only thiacloprid and PSMO activity have a high coefficient of determination (fig. 3, R 2 = 0.8989), showing that PSMO plays a role in thiacloprid detoxification in these species. The highest PSMO activity was observed for L. botrana females, the most tolerant group to thiacloprid (table 1, Navarro-Roldán et al., Reference Navarro-Roldán, Avilla, Bosch, Valls and Gemeno2017). Nevertheless, other detoxification mechanisms must be involved since the increase of thiacloprid toxicity detected with the application of the synergist PBO was not due to the increase of PSMO activity levels. A significant role of the PSMO in the detoxification of thiacloprid has been reported in C. pomonella field adults (Reyes et al., Reference Reyes, Franck, Charmillot, Ioriatti, Olivares, Pasqualini and Sauphanor2007), and in combination with EST in larvae (İşci & Ay, Reference İşci and Ay2017). The lack of correlation between enzymatic activity and mortality for the other insecticides and enzyme families may be due to lower insecticide selectivity and multiple target sites of the enzymatic groups resulting in mutual interactions (Ahmad & Hollingworth, Reference Ahmad and Hollingworth2004).
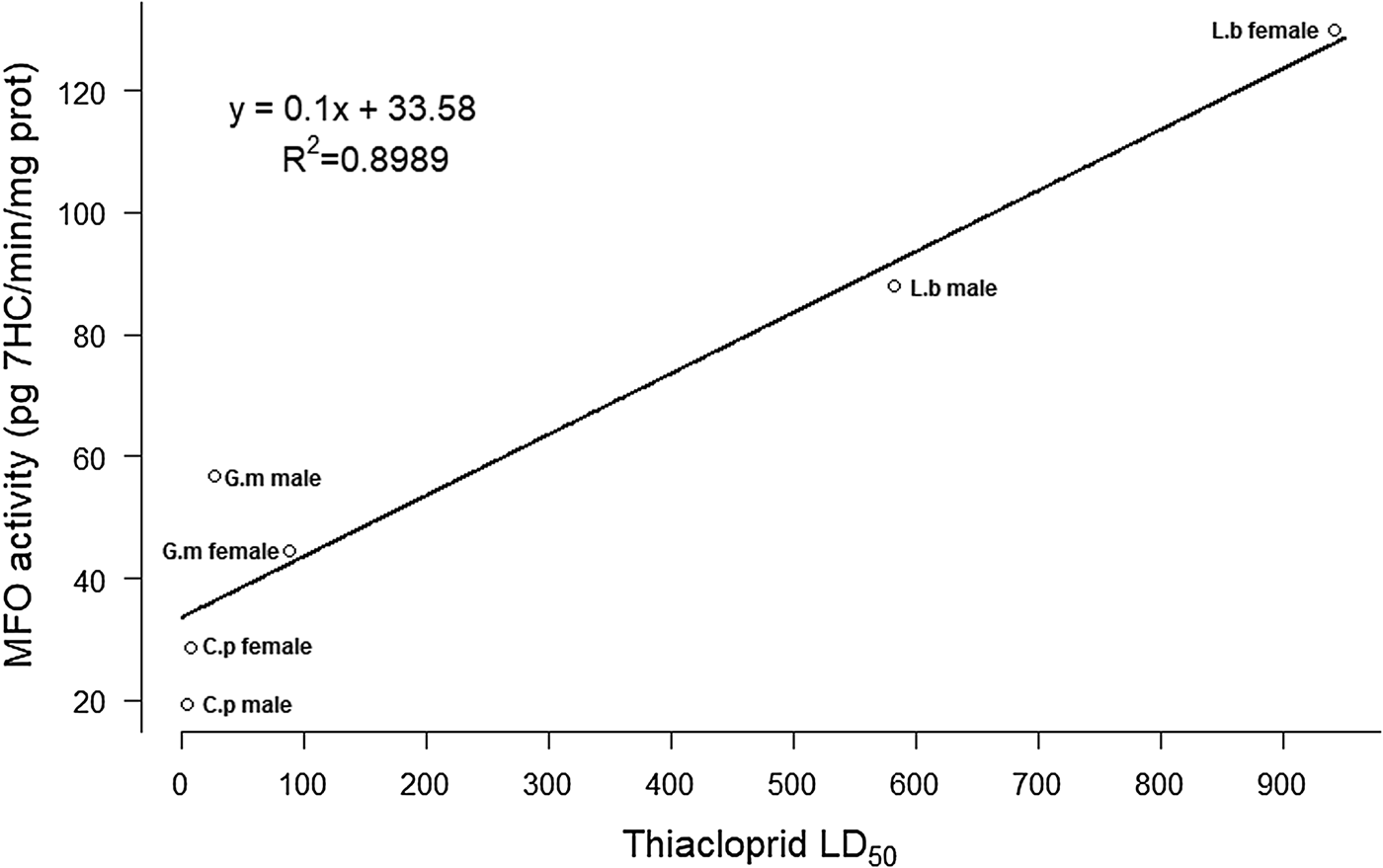
Fig. 3. Correlation between thiacloprid LD50 (ng of insecticide per mg of adult insect dry weight) (Navarro-Roldán et al., Reference Navarro-Roldán, Avilla, Bosch, Valls and Gemeno2017) and PSMO activity of acetone treated individuals (table 3). C.p: Cydia pomonella; G.m: Grapholita molesta; L.b: Lobesia botrana.
A remarkable finding from our previous study was lower susceptibility to the organophosphate insecticide chlorpyrifos in males of all three moth species compared to females, mainly in G. molesta (Navarro-Roldán et al., Reference Navarro-Roldán, Avilla, Bosch, Valls and Gemeno2017). The expected pattern was higher susceptibility in males due to their smaller size. Shearer & Usmani, (Reference Shearer and Usmani2001) reported higher female susceptibility to organophosphates in G. molesta. In a follow-up study, de Lame et al. (Reference de Lame, Hong, Shearer and Brattsten2001) reported that the higher tolerance of males may be linked to a higher EST and acetylcholinesterase (AChE) activity which may counteract the higher male AChE sensitivity to organophosphates. Contrarily, we have not found significant differences in EST activity between sexes, although a similar procedure was used in both studies. Our European laboratory strain was reared on an artificial diet for more than five years without reintroduction of wild individuals, whereas the North American population (de Lame et al., Reference de Lame, Hong, Shearer and Brattsten2001; Shearer & Usmani, Reference Shearer and Usmani2001) consisted of colonies, aged less than 3 years, reared on green apples. Thus, it is expected that both colonies possess very dissimilar genomes that condition their respective detoxification enzyme systems. Even an insect's feeding pattern, which can differ markedly during its breeding cycle, may influence its immune system (Vogelweith et al., Reference Vogelweith, Thiery, Quaglietti, Moret and Moreau2011). To further explain sex differences, de Lame et al. (Reference de Lame, Hong, Shearer and Brattsten2001) proposed a simultaneous involvement of other metabolic enzymatic families, like GST, or a lower bioactivation by PSMO of P = S compounds in the AChE inhibitory P = O analogs in males.
Conclusions
Differences in enzymatic activity quantification were found in the different studied species, being susceptible strains, reared for a long time in the laboratory and fed with the same artificial diet. L. botrana exhibited a general higher enzymatic activity, highlighting its high EST activity, and was the only species with differences in response between sexes for GST and PSMO activity. These enzymatic-activity differences could explain the lower susceptibility of females of this species to the insecticide products tested; however, they did not align with the sex differences reported in a previous study involving C. pomonella and G. molesta (Navarro-Roldán et al., Reference Navarro-Roldán, Avilla, Bosch, Valls and Gemeno2017).
As was showed by the mortality tests, the phase-I enzymatic activities (EST and PSMO) were involved in the insecticide detoxification in the three species for both sexes, highlighting the role of EST, whereas GST, phase-II enzymes, appeared to play less of a role in insecticide detoxification, resulting involved only in G. molesta insecticide detoxification. In addition, the positive correlation observed between PSMO activity and the thiacloprid LD50 explained the species-specific differences in susceptibility to thiacloprid that were reported in our previous study (Navarro-Roldán et al., Reference Navarro-Roldán, Avilla, Bosch, Valls and Gemeno2017).
The knowledge of the enzymatic activity level of susceptible populations of each species can be useful for susceptibility/resistant studies. The differentiation between sexes in L. botrana studies would be basic for a good characterization of the populations.
Acknowledgements
MAN-R was supported by a Ph.D. fellowship from the University of Lleida. This study was supported by the research Grant AGL2013-49164-C2-1- MINECO and AGL2016-77373-C2-2-R-MINECO, Spain. The authors wish to thank the CERCA Programme/Generalitat de Catalunya. Finally, our special appreciation goes to Jesús Avilla for his helpful comments on an earlier version of the manuscript, and to Sandrine Maugin for her laboratory collaboration.