Introduction
Helicoverpa armigera (Hübner) (Lepidoptera: Noctuidae) is a major pest of a wide range of field and horticultural crops in many parts of the world with permanent populations in most areas between 45°N and 45°S latitude (Hardwick, Reference Hardwick1965; Zalucki et al., Reference Zalucki, Daglish, Firempong and Twine1986, Reference Zalucki, Murray, Gregg, Fitt, Twine and Jones1994; Fitt, Reference Fitt1989). The wide distribution of this species shows that it can adapt to seasonal changes which affect its survival from wet–dry tropical to cool temperate regions (Qureshi et al., Reference Qureshi, Murai, Yoshida, Shiraga and Tsumuki1999; Mironidis et al., Reference Mironidis, Stamopoulos and Savopoulou-Soultani2010). This ability of H. armigera to tolerate climatic variation is derived, in part, from its four life-history characteristics: polyphagy, high mobility, high fecundity and a facultative diapause that enables the species to survive seasonally changing, and unstable habitats (Fitt, Reference Fitt1989).
Insect development occurs within a specific temperature range. Temperature influences the rates of growth and development of insects, the duration of life cycles, fecundity and survival (Howe, Reference Howe1967; Andrewartha, Reference Andrewartha1970). When investigating the effect of temperature on these parameters, the question arises as to whether constant or fluctuating temperatures should be used (Lamb, Reference Lamb1961). Most experimental studies of insect development and survival are conducted under constant temperature conditions, and the results from such studies usually provide the basis for an analysis of phenology and seasonal development in the field. These studies may produce misleading information on the ecophysiological impact of temperature on the population dynamics of a given species since in most natural environments, temperature usually undergoes regular diurnal variation, frequently superimposed by irregular fluctuations (Humpesch, Reference Humpesch1980; Behrens et al., Reference Behrens, Hoffmann, Kempa, Gäbler and Merkel-Wallner1983; Maharaj, Reference Maharaj2003; Carrington et al., Reference Carrington, Armijos, Lambrechts, Barker and Scott2013). Discrepancies are common between developmental times predicted from constant development–temperature relationships and those measured under natural fluctuating or alternating temperatures around the same mean (Mironidis & Savopoulou-Soultani, Reference Mironidis and Savopoulou-Soultani2008; Carrington et al., Reference Carrington, Armijos, Lambrechts, Barker and Scott2013). To mimic field conditions more realistically, development under 24 h cycling temperature regimes has been studied (for reviews see Ratte, Reference Ratte and Hoffman1985; Beck, Reference Beck, Lee and Denlinger1991; Liu et al., Reference Liu, Zhang and Zhu1995). Insects frequently develop more rapidly at fluctuating than at constant temperatures with equivalent means (Lamb, Reference Lamb1961; Hagstrum & Hagstrum, Reference Hagstrum and Hagstrum1970; Hagstrum & Leach, Reference Hagstrum and Leach1973; Beck, Reference Beck1983b ; Davis et al., Reference Davis, Radcliffe and Ragsdale2006; Fischer et al., Reference Fischer, Kölzow, Höltje and Karl2011). On the other hand, fluctuating temperatures not only cause specific accelerations, but they may also slow down development (Hagstrum & Milliken, Reference Hagstrum and Milliken1991; Petavy et al., Reference Petavy, David, Gibert and Moreteau2001). Finally, other results indicate that diurnally fluctuating temperatures have no effect on insect development (Hagstrum & Leach, Reference Hagstrum and Leach1973; Welbers, Reference Welbers1975; Humpesch, Reference Humpesch1982; Liu et al., Reference Liu, Zhang and Zhu1995). The intensity of these effects depends on the amplitude of fluctuating temperature regimes (Eubank et al., Reference Eubank, Atmar and Ellington1973; Lamb & Gerber, Reference Lamb and Gerber1985; Hagstrum & Milliken, Reference Hagstrum and Milliken1991; Petavy et al., Reference Petavy, David, Gibert and Moreteau2001; Carrington et al., Reference Carrington, Armijos, Lambrechts, Barker and Scott2013), on the insect species (stenothermic or eurythermic, see Hagstrum & Hagstrum, Reference Hagstrum and Hagstrum1970) and on the characteristics of the kinetics of temperature change (Hoffmann, Reference Hoffmann1980). According to Behrens et al. (Reference Behrens, Hoffmann, Kempa, Gäbler and Merkel-Wallner1983), it is not the length of warm treatment alone, but the temperature change per se, that further intensifies the temperature-induced modifications in development.
Several studies have been conducted on the effect of constant temperatures on growth parameters of H. armigera reared either on host-plant material or on artificial diet (Twine, Reference Twine1978; Kay, Reference Kay1981; Allsopp et al., Reference Allsopp, Daglish, Taylor, Gregg and Zalucki1991; Qureshi et al., Reference Qureshi, Murai, Yoshida, Shiraga and Tsumuki1999; Jallow & Matsumura, Reference Jallow and Matsumura2001; Liu et al., Reference Liu, Li, Gong and Wu2004; Bartekova & Praslicka, Reference Bartekova and Praslicka2006). The effect of alternating temperatures, which subjects insects to two different thermoperiods (thermophase–cryophase), has been also well documented (Foley, Reference Foley1981; Mironidis & Savopoulou-Soultani, Reference Mironidis and Savopoulou-Soultani2008, Reference Mironidis and Savopoulou-Soultani2012). Although there are several studies on the development of poikilothermic organisms subjected to gradually changing temperatures (fluctuating conditions) in the real diurnal cyclic variation (Lamb, Reference Lamb1961; Humpesch, Reference Humpesch1982; Hogg, Reference Hogg1985; Hagstrum & Milliken, Reference Hagstrum and Milliken1991; Maharaj, Reference Maharaj2003; Davis et al., Reference Davis, Radcliffe and Ragsdale2006; Fischer et al., Reference Fischer, Kölzow, Höltje and Karl2011; Carrington et al., Reference Carrington, Armijos, Lambrechts, Barker and Scott2013), there is a paucity of similar information in H. armigera. For this reason, it seems important to study the developmental rate for each life stage of this insect under fluctuating temperatures. As different life stages may inhabit different microhabitats, they may differ in their thermal sensitivities and other traits that are important for responses to climate (Kingsolver et al., Reference Kingsolver, Woods, Buckley, Potter, MacLean and Higgins2011). Consequently, such data are necessary to produce information on the ecophysiological impact of temperature on the population dynamics under field conditions.
The purpose of this study was to investigate the effects of fluctuating temperatures on development and survival of the immature stages of H. armigera, as well as the effects of temperature on demographic parameters (e.g. adult survival, longevity, fecundity and intrinsic rate of increase) under laboratory conditions. This information will lead to more appropriate phenological models for evaluation of pest management strategies and for population dynamics analysis and may contribute to a better understanding of the effects of climate change on H. armigera phenology.
Materials and methods
Insects
A laboratory colony of H. armigera was established in September 2010 from more than 400 larvae collected from cotton fields in Northern Greece (41°N, 023°E). The insects were reared on artificial diet and held at 25 °C, 60–70% RH, under a photoperiod 16:8 h (L:D). Maintenance and handling of the colony has been previously described (Mironidis & Savopoulou-Soultani, Reference Mironidis and Savopoulou-Soultani2008). The offspring of the F 5 generation were used to the experiments to reduce the possible influence of the host source.
Survival and development of immature stages
The effects of progressive change (stepwise change every hour) in temperature on survival and development of immature stages of H. armigera, were examined. Eggs laid on the same day (<24 h old) were kept in Petri dishes in controlled environment chambers (SANYO, MIR-154, SANYO Electric Co. Ltd, Osaka, Japan) and exposed to eight different fluctuating temperatures with mean value of 15–35 °C under constant photoperiodic conditions of 16:8 h (L:D) (photophase started at 8 am every day). Mean temperatures were selected to simulate the field temperature conditions prevailing from April to October in Northern Greece (fig. 1A) in which H. armigera completes its three to four generations per year (Mironidis, Reference Mironidis2009; Mironidis et al., Reference Mironidis, Stamopoulos and Savopoulou-Soultani2010). All temperatures tested had 24-h periods and ±9 °C amplitudes. The reported temperatures were accurate to within ±0.5 °C. Figure 1B depicts the curves of hourly temperatures through the 24-h cycle of fluctuating temperatures for mean temperatures of 15–35 °C. The transition from one temperature to another was essentially completed within approximately 1 min after switching. Light intensity in the chambers 22.5 μEin m−2 s−1 (400–700 nm) measures by a quantum sensor Li 188 B (LICOR Inc.). Relative humidity was approximately 60–65%.
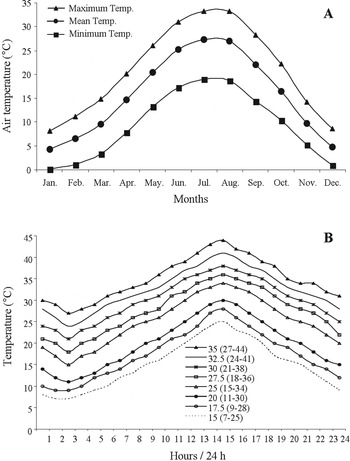
Fig. 1. (A) Mean monthly air temperature over 20 years (1989–2009) (Hellenic National Meteorological Service). (B) The hourly temperatures through the 24-h cycle of fluctuating temperatures for the mean temperatures of 15–35 °C. For each mean temperature, inside the parenthesis, is given the lower and upper temperature of the diurnal variation.
The number of eggs used to start each temperature treatment varied from 80 to 200 for most of the treatments. The number of eggs hatched was recorded daily, except for the eggs held at the higher mean temperatures (30, 32.5 and 35 °C), which were examined every 12 h. Larvae hatched from each temperature treatment were used for the developmental studies at the respective temperatures. Newly hatched first-instar larvae were individually transferred from the Petri dishes to 50 ml plastic cups and were provisioned with artificial diet. After the completion of the third instar the larvae were transferred to Petri dishes. Larvae were examined daily until pupation for moulting and mortality. Larval instars were determined by checking the shed head capsules (Mironidis, Reference Mironidis2009). Pupal weight was recorded on day 2 after pupation with an analytical balance (Chyo JK-180; ±0.01 mg; Chyo, Osaka, Japan). Upon pupation sex was determined and recorded. The number of emerged adults was recorded daily.
Adult longevity and reproduction
Adults emerged from the developmental study were used to estimate fecundity and adult longevity at the respective temperature treatments. Upon emergence (<24 h), pairs of adults were placed individually in transparent hard plastic truncated conical cups (400 ml) covered by a transparent piece of tulle. A hole punched in the bottom of each cup was plugged with a dental wick saturated with 10% sucrose solution as a food source. Adults were transferred to new cups daily until their death and eggs laid each day were counted under a binocular stereoscope (M26, 40×; Leica, Wetzlar, Germany). Preoviposition period, oviposition period and the number of eggs laid were determined.
Statistical analysis
The effect of fluctuating temperatures on developmental time and reproduction was determined by analysis of variance (ANOVA). The t-test was used to compare the values of the means from two samples. A logarithmic transformation log10(x+1) of the data was used to avoid heterogeneity of variance: untransformed means are presented in the tables. Percentages were compared using the Chi-square (χ2) test (Sokal & Rohlf, Reference Sokal and Rohlf1995).
The relationship between mean temperature (T) and mean developmental rate (r=developmental time−1) of each life stage and total immature stage (egg to adult emergence) under fluctuating temperatures was determined using linear and nonlinear models.
Linear model
The relationship between T and r was determined using linear regression where r=a+bT. The lower developmental threshold (T o) and the degree-days requirements (DD) were estimated as T o=−a/b and DD=1/b, where a and b were estimated by the least-square regression. Regressions were conducted only for those temperature ranges in which the developmental rate was linear with temperature. Calculations were performed with the statistical package STATISTICA (StatSoft Inc. 2001).
Nonlinear model
The nonlinear empirical Logan model (Logan et al., Reference Logan, Wollkind, Hoyt and Tanigoshi1976) as modified by Lactin et al. (Reference Lactin, Holliday, Johnson and Craigen1995) was used to describe the temperature-dependent developmental thresholds under fluctuating temperatures of each life stage and total immature stage (egg–adult). The expression of the model is as follows:

where r(T) is the mean developmental rate at temperature T (°C). Fitted parameters, ρ (the rate of increase at optimal temperature), T max (the upper developmental threshold), Δ (the difference between optimal and upper developmental thresholds) and λ (which allows the curve to intersect the x-axis, allowing the estimation of a developmental threshold), were estimated using the Marquardt algorithm in SPSS 14 (SPSS, 2006).
Life table parameter analysis
Life table studies were conducted for each fluctuating temperature as described by Carey (Reference Carey1993). Immature-stage survival data were obtained from the experiment described above. A sex ratio of 1:1 females/males (Mironidis, Reference Mironidis2009) was used for the development of the life table parameters. The following parameters were calculated: net reproductive rate (R o=ΣLx i mxi ), mean generation time (T=Σx i lximxi ), intrinsic rate of increase (r=lnR o/T), finite rate of increase (λ=anti-lnr), doubling time (DT=ln2/r), where lx i is the number of surviving insects at the beginning of age class x i , Lxi is the survivorship of age class x i and mx i is the number of female offspring produced per female in each age class x i . The average number of the offspring produced by each female in the interval x to x+1 (gross fecundity rate, M x ), was recorded until death.
Results
Survival and development of immature stages
H. armigera achieved complete development from egg to adult emergence between mean 17.5 and 32.5 °C at fluctuating temperatures examined (table 1). At mean 35 °C, 29% of eggs hatched successfully but all newly hatched larvae died; at mean 25 °C, the highest percentage of egg hatching was observed. At mean 15 °C, the insects entered diapause at the pupal stage. Lower mean temperatures (17.5 and 20 °C) caused less mortality than higher ones (30 and 32.5 °C). On the other hand, moderate mean temperatures (25 and 27.5 °C) were more favourable for H. armigera survival. Survival of the immature stages was significantly different among the temperature treatments, except for some larval instars (χ2 test, P<0.05) (table 1).
Table 1. Survivorship (percentage) of immature stages of H. armigera as a function of fluctuating temperatures.

1 Number of eggs used to start each of the temperature treatments.
2 Total represents survivorship of immature stages from egg to adult emergence.
3 Individuals exposed to mean 15 °C entered diapause at pupal stage.
Age-specific survivorship curves at fluctuating temperatures tested are depicted in fig. 2. Mortality rates remained approximately constant with age (type-II curve) at mean 17.5 to 27.5 °C (fig. 2b–e). At higher means the shape of the age-specific survivorship curve changes progressively to type III (Gutierrez, Reference Gutierrez1996; Price, Reference Price1997). This indicates that, above 30 °C, the death rate for H. armigera increased with most of the mortality occurring in early stages (fig. 2f–i).
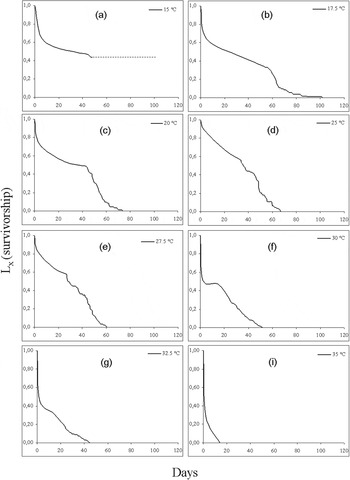
Fig. 2. Total survivorship (L x ) at fluctuating temperatures. The shift from smooth to a more ragged line in each graph depicts the transition from immature to adult stage. At mean 15 °C, the dotted line indicates the entrance in diapause at the pupal stage.
At fluctuating temperatures, the developmental times for egg stage of H. armigera decreased as temperature increased up to mean 32.5 °C, whereas in the larval and pupal stages the developmental rates became proportional to mean temperature (table 2). The duration of the larval stage decreased from 36.40 days at mean 15 °C to 11.96 days at mean 32.5 °C. Individuals exposed to mean 15 °C entered diapause at pupal stage and were therefore, not included in further analyses. The longer pupal duration was observed at mean 17.5 °C (19.78 days), while up to 27.5 °C the pupal duration was just over one-half this value (≈10 days). The total developmental time of immature stages varied inversely with temperature and ranged from 53.5 to 23.84 days at mean 17.5 and 32.5 °C, respectively. The mean pupal weight significantly decreased with the increase in the mean of fluctuating temperature. The highest pupal weight was observed at mean 15 °C (316.4 mg) and the lowest at 32.5 °C (225.3 mg) (table 2).
Table 2. Mean developmental time (days±SE) of immature stages and pupal weight (mg±SE) of H. armigera at fluctuating temperatures.

1 Total represents mean developmental time of immature stages from egg to adult emergence.
2 Means within a column followed by the same letter are not significantly different (P<0.05, Tukey's HSD test).
Modelling developmental rates
Based on these results, the lower threshold and degree-day requirement for the development of each immature life stage of H. armigera were estimated by the linear model (table 3). The ranges of fluctuating temperatures used in the regression analysis were 15–35, 15–32.5 and 17.5–32.5 °C for egg, larval and pupal stages, respectively. High coefficients of determination (R 2>0.9) were obtained for each immature stage and for the total immature stage (egg-to-adult emergence). The lower developmental thresholds (T o) were estimated to 7.69, 6.48 and 4.63 °C for egg, larval and pupal stages, respectively. The T o for development from egg to adult emergence was 6.13 °C. The required degree-days for egg, larval, pupal and the total immature stages development were 49.75, 303.03, 263.16 and 625, respectively (table 3).
Table 3. Linear regression model parameters and R 2 values for temperature-dependent developmental rates of H. armigera immature stages at fluctuating temperatures.

a, intercept; b, slope; T o, lower developmental threshold in °C; DD, cumulative degree-days required for stage development.
1 Total represents the development of immature stages from egg to adult emergence.
The nonlinear Lactin-2 model, when fitted to developmental rate values, gave a good fit to the data sets over the range of fluctuating temperature used (fig. 3; table 4). Observed developmental rate values for fluctuating temperatures were compared with predicted values from the Lactin-2 model for egg, larval, pupal and total immature (egg to adult emergence) stages (fig. 3). In the range of 17.5–32.5 °C, the responses obtained were approximately linear for all developmental stages. The resulting coefficients of determination were high (R 2>0.98) for all developmental stages (table 4). Lower values of developmental thresholds were estimated by the linear model than by the Lactin-2 model (tables 3 and 4). The optimal developmental temperatures estimated for egg, larval, pupal and total immature stages were 32.85, 32.25, 31.62 and 31.87 °C, respectively (table 4). Above the optimal temperature, developmental rate decreased. The upper developmental thresholds (t max) for each immature stage and for the total immature stage were estimated between 34.89 and 37.51 °C (fig. 3; table 4).

Fig. 3. Developmental rate (per day) of (a) egg, (b) larval, (c) pupal and (d) total immature (egg to adult emergence) stages of H. armigera as a function of fluctuating (●) temperature (°C). Fitted curves according to Lactin et al. (Reference Lactin, Holliday, Johnson and Craigen1995). Open circles (○) indicate developmental rates as a function of constant temperatures, after Mironidis & Savopoulou-Soultani (Reference Mironidis and Savopoulou-Soultani2008).
Table 4. Nonlinear (Lactin et al., Reference Lactin, Holliday, Johnson and Craigen1995) model parameters (±SE) and R 2 values for temperature-dependent developmental rates of H. armigera immature stages reared at fluctuating temperatures.

ρ, the rate of increase to optimum temperature; T max, lethal temperature; Δ, difference between the lethal temperature and the optimum temperature of development; λ, parameter that makes the curve intersect the x-axis; t min, t opt and t max, lower, optimum and maximum developmental threshold in °C.
1 Total represents the development of immature stages from egg to adult emergence.
Adult longevity and reproduction
Fluctuating temperatures significantly affected adult's longevity of both sexes. The average life span of male H. armigera ranged from 9.88 to 20.39 days, whereas of females from 11.09 to 18.56 days, at means 32.5 and 25 °C, respectively (table 5). Fluctuating temperatures produced different average adult lifespans; male lifespans were longer than females’ in most treatments; however, the differences were not significant (table 5).
Table 5. Reproductive parameters and longevity expectation of H. armigera at fluctuating temperatures.

1 Means within a column followed by the same letter (lower case) are not significantly different (P<0.05, Tukey's HSD test).
2 Means between sexes in a particular temperature followed by the same letter (upper case) are not significantly different (P<0.05, t-test).
The number of eggs produced by H. armigera females was affected by the fluctuating temperatures. The highest number of eggs was recorded at mean 25 °C (1130.47 eggs/female (♀)), whereas the lowest at mean 32.5 °C (427.09 eggs/♀). Fecundities for females reared between mean 20 and 30 °C were above the 1000 eggs/♀. The daily egg production ranged from 97.81 to 27.47 eggs at mean 27.5 and 32.5 °C, respectively (table 5). The number of eggs laid per female per day (M x ) was approximately similar at moderate fluctuating temperatures (fig. 4b–d), whereas the M x decreased at high fluctuating temperatures (fig. 4f).

Fig. 4. Number of eggs per female per day (M x ) under fluctuating temperatures (●). Open circles (○) indicate fecundity occurring under constant temperatures, after Mironidis & Savopoulou-Soultani (Reference Mironidis and Savopoulou-Soultani2008).
The duration of the preoviposition period of H. armigera was also affected by the temperature. It was highest at mean 17.5 °C (2.01 days) and lowest at mean 27.5 °C (0.82 day). Females underwent a distinct oviposition period that varied with the fluctuating temperature. The longest oviposition period (15.67 days) was recorded at mean 25 °C and the shortest (8.40 days) at mean 17.5 °C.
Life table parameters
The estimated life table parameters for H. armigera at different fluctuating temperatures are presented in table 6. The highest value of net reproductive rate (R o) was estimated for insects reared at mean 25 °C, whereas the lowest at mean 32.5 °C. The intrinsic (r) and finite (λ) rates of increase were highest at mean 27.5 °C. Increasing mean temperature resulted in shorter mean generation times. The longest population doubling time occurred at mean 17.5 °C.
Table 6. Summary of life table parameters of H. armigera at fluctuating temperatures.

Discussion
In natural conditions, organisms are subjected to a combination of environmental factors, both biotic and abiotic, and it is this combination that ultimately determines the distribution and abundance of a species (Gillott, Reference Gillott2005). It is well known that the majority of insects, as of many other organisms experience daily cycles of light and dark, seasonal climate cycles, cycles of feeding (correlated with the presence or the absence of food) and temperature or humidity cycles, which, in many cases, regulate both their behaviour and their physiology (Stamopoulos, Reference Stamopoulos1989; Mironidis et al., Reference Mironidis, Stamopoulos and Savopoulou-Soultani2010), and whose influence on development may be somewhat different from that of constant conditions (Beck, Reference Beck1980, Reference Beck1983a , Reference Beck b ; Mironidis & Savopoulou-Soultani, Reference Mironidis and Savopoulou-Soultani2008). To cope with this environmental variation insects have evolved a variety of alternative developmental pathways (phenotypic plasticity) (Tauber et al., Reference Tauber, Tauber and Masaki1986; Johansson et al., Reference Johansson, Stoks, Rowe and De Block2001; Grimaldi & Engel, Reference Grimaldi and Engel2005; Fischer et al., Reference Fischer, Kölzow, Höltje and Karl2011).
The upper lethal temperature for the majority of insects, being exposed for a brief time period (∼1 h) to high temperatures, is located in the range of 40–50 °C (Bursell, Reference Bursell and Rockstein1964). The fluctuating temperature with mean 35 °C and diurnal range of 9 °C did not support development to maturity; 29% of eggs hatched successfully but all newly hatched larvae died since insects were exposed for some time at temperatures ranging from 40 to 44 °C (fig. 1B), which were deleterious for their survival (fig. 2i, table 1). This result is consistent with that of Singh & Lakhotia (Reference Singh and Lakhotia2000) who found that the exposure of fifth-instar larvae of H. armigera at 45 °C for 40 min was lethal for most insect tissues. Howe (Reference Howe1967) noted that outside the survival range, the effects of temperature on survival and development were confounded with time of exposure. In natural conditions, many insects experience changes in temperature on timescales of a few hours, and are able to invoke physiological responses to aid survival or maintain other activities, such as movement or flight (Bale & Hayward, Reference Bale and Hayward2010). Consequently, the magnitude of temperature fluctuations as well as the mean temperature around which they oscillate are likely to alter the direction of the effects of changing conditions on insect life-history parameters (Andrewartha, Reference Andrewartha1970; Petavy et al., Reference Petavy, David, Gibert and Moreteau2001; Carrington et al., Reference Carrington, Armijos, Lambrechts, Barker and Scott2013). Furthermore, Kingsolver et al. (Reference Kingsolver, Ragland and Diamond2009) suggest that mean and extreme temperatures may act as more potent selective forces on thermal reaction norms than temperature variation per se. As climate change will not only alter mean temperatures, but also the daily temperature ranges (Easterling et al., Reference Easterling, Meehl, Parmesan, Changnon, Karl and Mearns2000; Vose et al., Reference Vose, Easterling and Gleason2005); understanding these effects is necessary to define the ‘realized’ thermal reaction norms (i.e. the actual fitness curves observed under variable conditions in nature) for different species and to quantify vulnerability to climate warming (Paaijmans et al., Reference Paaijmans, Heinig, Seliga, Blanford, Blanford, Murdock and Thomas2013).
Survival of H.armigera reared under 24-h fluctuating temperature cycles with ±9 °C amplitudes over a wide thermal range, mean 17.5 up to mean 27.5 °C, is almost stable and apparently not affected by temperature. Below 17.5 °C, insects exposed to mean 15 °C entered diapause in the pupal stage. At warmer mean temperatures, survival decreased very quickly above 28 °C and fell to zero at mean 35 °C (table 1, fig. 2). Mironidis & Savopoulou-Soultani (Reference Mironidis and Savopoulou-Soultani2008) showed that H. armigera, when reared at constant temperatures, could only develop from egg to adult emergence (capable of egg production) between 17.5 and 32.5 °C, but fluctuating temperatures allowed H. armigera to complete its life cycle over a much wider range, 9 to 41 °C (table 1 and fig. 1B). However, complete development was possible for some individual instars and stages at temperatures below or above this range. Moreover, different stages varied in their temperature limits for complete development, with the egg stage having the widest range (6–44 °C) (table 1). Similarly, Liu et al. (Reference Liu, Chen and Zalucki2002) found that Plutella xylostela (L.) (Lepidoptera: Plutellidae) developed successfully from egg to adult emergence at constant temperatures from 8 to 32 °C and under changing temperature regimens, including temperatures as low as 4 °C or as high as 38 °C. The fluctuating temperatures resulted in higher total survival rates of H. armigera compared to those of corresponding constant temperatures that we previously estimated (Mironidis & Savopoulou-Soultani, Reference Mironidis and Savopoulou-Soultani2008), probably because fluctuating temperatures provide recovery time or permit adaptation at extreme hot or cold temperatures (Vargas et al., Reference Vargas, Walsh, Kanehisa, Stark and Nishida2000). Dhileepan et al. (Reference Dhileepan, Trevino and Raghu2005) found that the survival of Aconophora coppressa (Walker) at fluctuating temperatures was higher than under constant temperatures, but the general trend of lower survival at higher temperatures was similar to that found in our study. In the same way, Davis et al. (Reference Davis, Radcliffe and Ragsdale2006) showed that fluctuating temperatures enhance survivorship of green peach aphid Myzus persicae (Sulzer) (Hemiptera: Aphididae) at the lowest and highest temperature regimens in relation to constant conditions and attributed these results to the fact that fluctuating temperatures more closely model what occurs in nature. Bursell (Reference Bursell and Rockstein1964) claimed that a process of physiological acclimation may take place when the insects are exposed to sublethal temperatures before they are exposed to critical ones. This process enables the insects to survive at critical temperatures.
It has often been suggested that fluctuating temperatures have some special stimulating effect on insect development when compared to development at constant temperatures with equivalent means. Hagstrum & Hagstrum (Reference Hagstrum and Hagstrum1970) and Hagstrum & Milliken (Reference Hagstrum and Milliken1991) have summarized a diversity of papers on this subject. In our results, the total developmental time of immature stages of H. armigera varied inversely with mean fluctuating temperature and ranged from 53.5 to 23.84 days at mean 17.5 and 32.5 °C, respectively (table 2). These developmental times tended to be shorter at low fluctuating temperatures and longer at higher ones compared to developmental times at constant temperatures with the same means (fig. 3; Mironidis & Savopoulou-Soultani, Reference Mironidis and Savopoulou-Soultani2008). The differences in developmental times between constant and fluctuating conditions were much more pronounced at the cooler compared to the warmer mean temperatures, whereas little or no difference was observed at intermediate temperatures. Specifically, at 15 °C, despite the fact that at both fluctuating and constant conditions insects entered diapause in the pupal stage, the larval developmental time was prolonged for 32 days at constant (68.00 days) (Mironidis & Savopoulou-Soultani, Reference Mironidis and Savopoulou-Soultani2008) as compared to fluctuating conditions (36.40 days). In the same way, other authors (Behrens et al., Reference Behrens, Hoffmann, Kempa, Gäbler and Merkel-Wallner1983; Lamb & Gerber, Reference Lamb and Gerber1985; Roltsch et al., Reference Roltsch, Mayse and Clausen1990; Hagstrum & Milliken, Reference Hagstrum and Milliken1991; Bryant et al., Reference Bryant, Bale and Thomas1999; Petavy et al., Reference Petavy, David, Gibert and Moreteau2001; Fantinou et al., Reference Fantinou, Perdikis and Chatzoglou2003; Mironidis & Savopoulou-Soultani, Reference Mironidis and Savopoulou-Soultani2008; Fischer et al., Reference Fischer, Kölzow, Höltje and Karl2011) have confirmed this acceleration in developmental time at low to moderate changing temperature conditions relative to corresponding constant ones. Species differences between developmental times at constant temperatures and those at fluctuating ones are partially a function of the ranges of temperatures and amplitudes of fluctuations used in different studies (Hagstrum & Hagstrum, Reference Hagstrum and Hagstrum1970; Hagstrum & Milliken, Reference Hagstrum and Milliken1991; Fischer et al., Reference Fischer, Kölzow, Höltje and Karl2011).
It is generally accepted that the acceleration of insect development at low fluctuating temperatures and the retardation at higher ones is a consequence of the nonlinearity inherent in the temperature–developmental rate relationship (Keen, Reference Keen1979; Behrens et al., Reference Behrens, Hoffmann, Kempa, Gäbler and Merkel-Wallner1983; Bryant et al., Reference Bryant, Bale and Thomas1999; Fantinou et al., Reference Fantinou, Perdikis and Chatzoglou2003; Vangansbeke et al., Reference Vangansbeke, De Schrijver, Spranghers, Audenaert, Verhoeven, Nguyen, Gobin, Tirry and De Clercq2013). It was first described by Kaufmann (Reference Kaufmann1932) and is known as the Kaufmann (Worner, Reference Worner1992) or rate summing effect (Ratte, Reference Ratte and Hoffman1985). Some studies invoke additional physiological mechanisms or specific adaptations such as thermoperiodic stimulation of the neuroendocrine system (Sharpe & DeMichele, Reference Sharpe and DeMichele1977; Beck, Reference Beck1983b ), circadian effects and optimization and/or stabilization of enzyme functioning (Ratte, Reference Ratte and Hoffman1985; Rock, Reference Rock1985; Fields, Reference Fields2001) to explain the alteration of developmental rates under fluctuating conditions. Moreover, insects living in a temperature habitat, which fluctuates with a daily rhythm organize their activities (such as feeding and resting), and metabolic processes, in accordance with the diurnally rhythmic change of atmospheric temperature (Behrens et al., Reference Behrens, Hoffmann, Kempa, Gäbler and Merkel-Wallner1983); increased food intake during daytime owing to favourably high temperatures, but decreased metabolic losses during the cooler night time (Brakefield & Mazzotta, Reference Brakefield and Mazzotta1995; Fischer et al., Reference Fischer, Kölzow, Höltje and Karl2011). Nevertheless, we cannot dismiss the possibility that constant temperatures may have direct, undesirable effects on insect metabolic processes (Lamb, Reference Lamb1961). Another aspect for consideration regarding the acceleration of development is the thermal units accumulated when the fluctuating temperature oscillates below developmental threshold, compared to the thermal units accumulated at low constant temperatures (Yeargan, Reference Yeargan1980). Calculations by Richards & Suanraksa (Reference Richards and Suanraksa1962) showed that the energy expenditure for embryonic development of Oncopellus fasciatus (Dallas) (Hemiptera: Lygaeidae) at changing temperature conditions was less than that needed at corresponding constant temperatures. They also showed that the energy reserves were sufficient for considerable periods below the constant temperature threshold, provided that enough time was spent at much higher temperatures. On the other hand, the oscillation of high fluctuating temperatures exposes insects to extremely high temperatures (>40 °C) for brief time periods (fig. 1B). This heat stress alters insects fitness parameters (Mironidis & Savopoulou-Soultani, Reference Mironidis and Savopoulou-Soultani2010), and probably explains the retardation of H. armigera developmental rate at high fluctuating temperatures.
The results obtained from constant temperature experiments are often not applicable directly to the field (Lamb, Reference Lamb1961; Humpesch, Reference Humpesch1980) where insects are subjected to diurnal variation of temperature and such information need to be validated under fluctuating conditions before using for predictive purpose in the field (Carrington et al., Reference Carrington, Armijos, Lambrechts, Barker and Scott2013). Fluctuating temperatures approach more realistically the variation existing in nature and produce higher parameter values than the optimal constant temperature (Welbers, Reference Welbers1975; Behrens et al., Reference Behrens, Hoffmann, Kempa, Gäbler and Merkel-Wallner1983; Saffar et al., Reference Saffar, Grainger and Aldrich1996; Vargas et al., Reference Vargas, Walsh, Kanehisa, Stark and Nishida2000; Davis et al., Reference Davis, Radcliffe and Ragsdale2006). However, Roltsch et al. (Reference Roltsch, Mayse and Clausen1990) suggest that development within the context of diurnal temperature fluctuations does not result in a physiological change causing an immediate reduction in growth rate. The data from our study fit the linear and Lactin-2 models very well as indicated by the high R 2 values (tables 3 and 4). The estimate of λ value for all cases was <0, indicating that a developmental threshold can be estimated by the Lactin-2 model (Lactin et al., Reference Lactin, Holliday, Johnson and Craigen1995). The estimated upper development thresholds were close to the temperatures tested at which high mortality rates were observed (fig. 2i, table 4). The lower developmental threshold values for the egg, larval, pupal and total immature stages estimated by the linear model were slightly lower than those estimated by Lactin-2 model (tables 3 and 4). Roltsch et al. (Reference Roltsch, Mayse and Clausen1990) claim that the error involved in nonlinear, temperature-dependent developmental models may relate to the critical assumption that enzyme systems respond almost instantaneously to temperature changes.
Fluctuating temperatures are normal for many insect species: they show differing abilities to withstand constant temperature since the thermal optimum in fluctuating temperature may differ from the constant temperature optimum (Cloudsley-Thompson, Reference Cloudsley-Thompson1953). Messenger (Reference Messenger1964) reported that constant temperature studies underestimated thresholds, whereas fluctuating temperatures widened threshold limits. The lower developmental thresholds estimated via the linear model for egg (7.69 °C), larval (6.48 °C) and pupal (4.63 °C) stages under fluctuating temperatures in the present study were lower compared to those estimated by several authors under constant temperatures for laboratory strains of H. armigera reared on artificial diet or plant material for egg (8.61–14.8 °C), larval (10.52 to 11.7 °C) and pupal (8.2–14.8 °C) stages, respectively (Twine, Reference Twine1978; Wilson et al., Reference Wilson, Lewis and Cunningham1979; Foley, Reference Foley1981; Kay, Reference Kay1981; Qureshi et al., Reference Qureshi, Murai, Yoshida, Shiraga and Tsumuki1999; Jallow & Matsumura, Reference Jallow and Matsumura2001; Bartekova & Praslicka, Reference Bartekova and Praslicka2006; Mironidis & Savopoulou-Soultani, Reference Mironidis and Savopoulou-Soultani2008; Fathipour & Sedaratian, Reference Fathipour and Sedaratian2013). Differences between the results obtained in this study and those of other studies could be attributed to the different origin of H. armigera, i.e. strain, host, geographical region, as well as to the different experimental conditions, i.e. rearing techniques. Insect response to variable temperatures, whether linear or nonlinear, can explain the shift in thresholds often observed for insect development. For example, Behrens et al. (Reference Behrens, Hoffmann, Kempa, Gäbler and Merkel-Wallner1983) found that changing temperatures with an amplitude of 12 °C reduced the temperature threshold for embryonic development of the cricket, Gryllus bimaculatus (De Geer) (Orthoptera: Gryllidae) from 16.6 °C at constant temperatures to 11.8 °C. Similarly, Fantinou et al. (Reference Fantinou, Perdikis and Chatzoglou2003) estimated lower developmental thresholds of the various stages of the corn borer, Sesamia nonagrioides (Lefebvre) (Lepidoptera: Noctuidae) when the insects were exposed to changing temperatures (threshold range: 6.2–7.2 °C) than when they were exposed to constant temperatures (threshold range: 8.9–10.8 °C).
In our study, as mean temperature increased, the duration of adult life span gradually increased, reaching the maximum at mean 25 °C. The further increase in the mean temperature progressively declined adult longevity, which was minimum at the highest fluctuated temperature examined (table 5). In all fluctuating temperatures tested the adults’ longevity of both sexes was lower than this recorded under constant conditions by Mironidis & Savopoulou-Soultani (Reference Mironidis and Savopoulou-Soultani2008), with the exception of females reared at 32.5 °C, with the differences to be more profound at the lower temperatures. The capacity of H. armigera to produce progeny, as measured by the mean total fecundity of females, reflects response pattern similar to that for adult longevity (table 5 and fig. 4). All fluctuating temperatures caused females to oviposit more eggs than they are able to under the corresponding constant temperatures (fig. 4). The minimum reproductive performances under fluctuating conditions, 427 and 708 eggs per female at mean 32.5 and 17.5 °C, respectively (table 5), were 33 and 44% greater than that recorded under constant conditions with equivalent means. However, the maximum oviposition under fluctuating conditions (1130 eggs per female) was observed at mean 25 °C and did not differ significantly compared to corresponding constant one (1007 eggs per female, Mironidis & Savopoulou-Soultani, Reference Mironidis and Savopoulou-Soultani2008) (fig. 4). In the same way, Davis et al. (Reference Davis, Radcliffe and Ragsdale2006) found that the green peach aphid developed faster and had greater fecundity under fluctuating conditions. Welbers (Reference Welbers1975) also stated that the diurnally changing temperatures stimulated the oviposition of Pectinophora gossypiella (Saunders) (Lepidoptera: Gelechiidae); the number of eggs per female was nearly twice the number as compared with the corresponding constant temperatures. On the contrary, Carrington et al. (Reference Carrington, Armijos, Lambrechts, Barker and Scott2013) have shown that the upper and lower ends of the fluctuating temperature scale have negative impact on reproduction of Aedes aegypti (Linnaeus) (Diptera: Culicidae) while Hagstrum & Leach (Reference Hagstrum and Leach1973) reported that changing conditions, compared to constant ones, had no effect on the number of produced eggs of Trogoderma inclusum LeConte (Coleoptera: Dermestidae) and Sitophilus oryzae (L.) (Coleoptera: Curculionidae).
The intrinsic rate of population increase, computed from life table data, is a good bioclimatic index, in that it assembles several life table parameters such as age-specific survivorship, developmental time and reproduction of the population into one value (Messenger, Reference Messenger1964). In our results, this index is positive, meaning that H. armigera could be expected to persist or increase in number between 9 and 41 °C (mean 17.5–32.5 °C), with a maximum value at mean 27.5 °C (0.17). This high r-value at this fluctuating temperature could be attributed to the considerably higher net reproductive rate per female (R o=351.04) and lower mean generation time (T=34.40) (table 6). Fluctuating temperatures resulted in higher r-values than that occurred at corresponding constant temperatures (Mironidis & Savopoulou-Soultani, Reference Mironidis and Savopoulou-Soultani2008), but at both conditions the optimum temperature, on the basis of intrinsic rate of increase, was apparently 27.5 °C. Similarly, other authors (Kieckhefer & Elliott, Reference Kieckhefer and Elliott1989; Vargas et al., Reference Vargas, Walsh, Kanehisa, Stark and Nishida2000; Davis et al., Reference Davis, Radcliffe and Ragsdale2006) found higher intrinsic rates of population increase for different insect species under changing temperatures. Changing temperature conditions result in higher r-values than do constant ones, because of shorter mean generation time as well as higher oviposition and reproduction (Kieckhefer & Elliott, Reference Kieckhefer and Elliott1989). Andrewartha (Reference Andrewartha1970) stated that the range of temperature favourable to a particular species is related to the prevailing temperatures in the places where the animals usually live. Cleary, this is true for H. armigera, which is of cosmopolitan distribution (Fitt, Reference Fitt1989) and as indicated by our results, able to survive and reproduce under a wide range of low-to-high fluctuating temperature conditions.
Many organisms live in variable thermal environments, which pose substantial challenges to survival and reproduction. Our results showed that H. armigera survives, develops and reproduces within a wide range of fluctuating temperatures (9–41 °C), while it completes the above functions with different levels of success at different mean temperatures of diurnal variation. Under field conditions in Northern Greece, the temperature fluctuates frequently. The temperature difference between day and night is often greater than 15 °C (Mironidis, Reference Mironidis2009). The daily average temperature in locations where H. armigera lives in Greece rarely reaches 30 °C (fig. 1A), but the maximum daily air temperatures during the summer, routinely exceed 40 °C, with extremes up to 45 °C (Mironidis & Savopoulou-Soultani, Reference Mironidis and Savopoulou-Soultani2010). Understanding mechanisms by which animals respond to environmental variation has taken on new urgency, due to increasing effects of global warming and climate change on natural systems (Huey & Kingsolver, Reference Huey and Kingsolver1989). This study investigated the effects of fluctuating temperatures on survival, development and on life history parameters of H. armigera life stages. This information is essential to understand the ecological and evolutionary consequences of climate change and can be used to develop a more profound understanding of H. armigera phenology under natural conditions. The results have implications for predicting population dynamics, distribution and dispersal of this insect and also for developing sustainable and integrated strategies for its management.