Introduction
Bunger Hills are located on the eastern flank of Denman and Scott glaciers (Fig. 1), one of the larger glacier systems in East Antarctica that influence mass balance of the ice sheet (Rignot et al. Reference Rignot, Mouginot, Scheuchl, van den Broeke, van Wessem and Morloghem2019). Although unglaciated coastal areas are not directly affected by changes in the larger glacier systems, their relief and sediments provide important paleogeographic information for understanding postglacial changes in climate, sea level and glacial extent (e.g. Gore et al. Reference Gore, Rhodes, Augustinus, Leishman, Colhoun and Rees-Jones2001, Verleyen et al. Reference Verleyen, Hodgson, Sabbe, Cremer, Emslie and Gibson2011, Berg et al. Reference Berg, White, Bennike, Fülöp, Fink, Wagner and Melles2016, Hodgson et al. Reference Hodgson, Whitehouse, DeCort, Berg, Verleyen, Tavernier, Roberts, Vyverman, Sabbe and O'Brien2016).
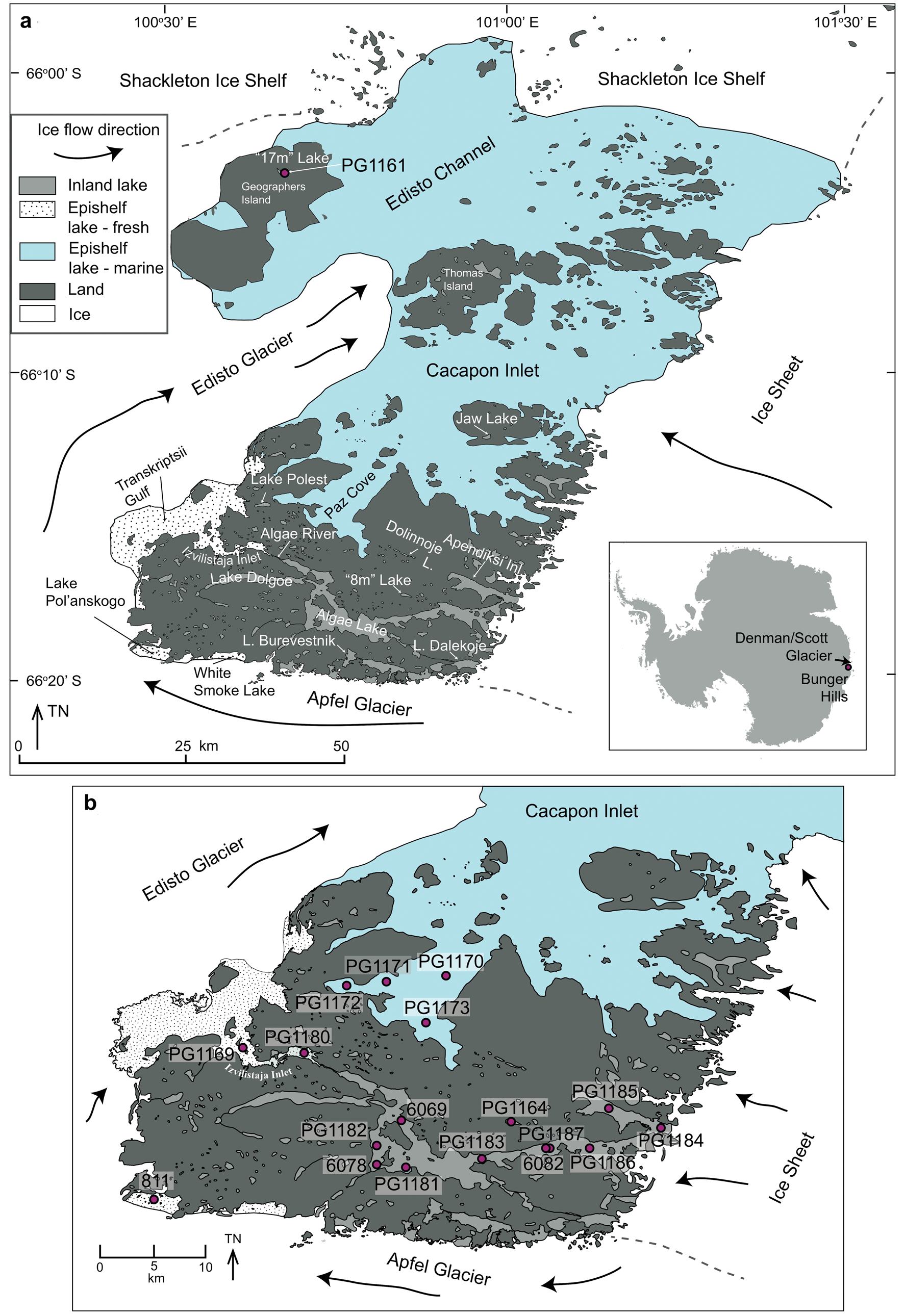
Fig. 1. Bunger Hills, a. place names mentioned in the text, and b. coring locations in the southern Bunger Hills. The coring location PG1161 on Geographers Island is shown in a.
Exposure of Bunger Hills as nunataks from the ice sheet started some time prior to 30 ka bp (Gore et al. Reference Gore, Rhodes, Augustinus, Leishman, Colhoun and Rees-Jones2001). The presence of unglaciated terrain during the Last Glacial Maximum (20–18 ka bp) makes Bunger Hills one of a number of potential refuges for Antarctic biota during periods of expanded ice sheets, glaciers and sea ice (e.g. Gibson et al. Reference Gibson, Paterson, White and Swadling2009, Berg et al. Reference Berg, White, Bennike, Fülöp, Fink, Wagner and Melles2016). Optically Stimulated Luminescence (OSL) ages of glaciofluvial and glaciolacustrine sediments reveal gradual downwasting of ice and expansion of nunatak area by 20 ka bp, and drainage of most glacial lakes by the beginning of the Holocene (Gore et al. Reference Gore, Rhodes, Augustinus, Leishman, Colhoun and Rees-Jones2001).
Most studies agree on a relatively stable position of the ice sheet margin throughout the Holocene (Verkulich & Hiller Reference Verkulich and Hiller1994, Fitzsimons & Colhoun Reference Fitzsimons and Colhoun1995, Doran et al. Reference Doran, Wharton, Lyons, Des Marais and Andersen2000). However, glaciers to the south-west of Bunger Hills fluctuated in extent (Colhoun & Adamson Reference Colhoun and Adamson1992, Augustinus Reference Augustinus2002) and grounding line position (Melles et al. Reference Melles, Kulbe, Verkulich, Pushina, Hubberten and Ricci1997). The timing of these advances and retreats is not well constrained. More precise determination of the timing and extent of glacier fluctuations could, however, give important insights into the sensitivity of the drainage system to climatic changes and oceanic forcings (Crosta et al. Reference Crosta, Crespin, Swingedouw, Marti, Masson-Delmotte, Etourneau, Goosse, Braconnot, Yam, Brailovski and Shemesh2018). Isostatic rebound and relative sea level (RSL) is an indirect measure of regional ice loading and deglaciation history (e.g. Hodgson et al. Reference Hodgson, Whitehouse, DeCort, Berg, Verleyen, Tavernier, Roberts, Vyverman, Sabbe and O'Brien2016). Estimates of the height of a mid-Holocene RSL high stand in Bunger Hills range from 7.5 ± 0.5 m (Colhoun & Adamson Reference Colhoun and Adamson1992) to 9–11 m (Roberts et al. Reference Roberts, McMinn and Zwartz2000, Verkulich et al. Reference Verkulich, Pushina, Sokratova, Melles, Hultsch and Diekmann2007). Holocene changes in RSL were inferred from diatom records from epishelf lakes (Verkulich et al. Reference Verkulich, Pushina, Sokratova, Melles, Hultsch and Diekmann2007). Maximum occurrence of open-ocean diatom species in the mid-Holocene as well as an increase in brackish and benthic species since the mid-Holocene point to a decrease in water exchange with the open ocean and decrease in water depth, probably reflecting RSL lowering (Verkulich et al. Reference Verkulich, Pushina, Sokratova, Melles, Hultsch and Diekmann2007).
The Holocene temperature development in Bunger Hills was deduced from the amount of organic matter deposition in the water bodies and the presence of sea ice diatoms in marine sediments. In the early Holocene, widespread deposition of biogenic sediments started in the water bodies of southern Bunger Hills indicating relatively warm conditions (e.g. Bolshiyanov et al. Reference Bolshiyanov, Verkulich, Klokov and Arslanov1991, Melles et al. Reference Melles, Verkulich and Hermichen1994a, Kulbe Reference Kulbe1997, Melles et al. Reference Melles, Kulbe, Verkulich, Pushina, Hubberten and Ricci1997, Kulbe et al. Reference Kulbe, Melles, Verkulich and Pushina2001, Verkulich et al. Reference Verkulich, Melles, Hubberten and Pushina2002). A climate optimum from 3.5 to 2.5 ka bp was inferred from high accumulation rates and δ13C values of organic carbon in a marine record from Paz Cove (also called Fishtail Bay and Rybiy-Khvost Bay, Fig. 1, Kulbe et al. Reference Kulbe, Melles, Verkulich and Pushina2001). Warmer water temperatures are also indicated by the diatom assemblage from the same record (Verkulich Reference Verkulich2007), however, reduced proportions of sea ice diatoms from 4.3–1.8 ka bp indicate a different timing than suggested from the geochemical data. Both proxies reflect a subsequent cooling and more variable conditions in the late Holocene. Records from Algae Lake (also called Figurnoye Lake) are more sensitive to changes in air temperature, which controls lake ice coverage and water balance of the lake. A multiproxy study on sediment records from Algae Lake showed that cooler conditions characterized the period from 9.0 ± 0.5 to 5.5 ± 0.5 ka bp and also during the late Holocene (Verkulich et al. Reference Verkulich, Melles, Hubberten and Pushina2002).
In this paper we review lithological, geochemical and diatom assemblage data from sediment records from three inland lakes and two epishelf lakes in Bunger Hills (Fig. 1, Table 1) that were previously presented and discussed by Melles et al. (Reference Melles, Verkulich and Hermichen1994a, Reference Melles, Kulbe, Verkulich, Pushina, Hubberten and Ricci1997), Kulbe (Reference Kulbe1997), Verkulich et al. (Reference Verkulich, Melles, Hubberten and Pushina2002), and Verkulich et al. (Reference Verkulich, Pushina, Sokratova, Melles, Hultsch and Diekmann2007). Some of the data has been presented in German and Russian publications (Kulbe Reference Kulbe1997, Verkulich et al. Reference Verkulich, Pushina, Sokratova, Melles, Hultsch and Diekmann2007) and are now made available to a broader audience. Based on new age-depth models for well-dated sediment cores we discuss evidence for the timing of initial deglaciation and subsequent fluctuations of the ice sheet margin, grounding-line positions of adjacent glaciers and evidence for RSL evolution and temperature. Our re-evaluation of existing data focuses on identifying depositional environments (marine or lacustrine) and transitions between them, which may indicate RSL changes and/or shifts in grounding line related to glacier advances.
Table I. Cores, coring locations and reference to records discussed in the text.

FW = fresh water, inland lake, ES = epishelf lake
Study site
Cacapon Inlet and Paz Cove in central Bunger Hills are presently filled with marine waters and host a rich marine benthic and planktonic flora and fauna (Klokov et al. Reference Klokov, Kaup, Zierath and Haendel1990, Melles et al. Reference Melles, Kulbe, Overduin and Verkulich1994b). A connection with the ocean (evidenced by tidal movement) also exists for epishelf lakes along the margins of Edisto Glacier and western Apfel Glacier, but these lakes today exhibit a freshwater layer due to restricted water exchange underneath the glacier (Doran et al. Reference Doran, Wharton, Lyons, Des Marais and Andersen2000). Transkriptsii Gulf is fed by water flowing from Algae Lake and from direct input of glacier melt from Apfel and Edisto glaciers (Fig. 1), resulting in stable stratification of the water column with fresh water overlying saline and anoxic waters below 88 m (Klokov et al. Reference Klokov, Kaup, Zierath and Haendel1990).
In addition to epishelf lakes, numerous inland lakes and ponds are distributed across Bunger Hills. Lakes at low altitudes (< 10 m above sea level, a.s.l.) are mostly saline and their water properties point to an origin as isolated marine basins, inheriting a sea-water signature in their ionic composition (e.g. Lake Polest, Fig. 1; Kaup et al. Reference Kaup, Haendel and Vaikmäe1993, Gibson et al. Reference Gibson, Roberts and Van de Vijer2006). Lakes that are fed by meltwater from the ice sheet or from glaciers show very low electrical conductivities. Low chlorophyll and phytoplankton concentrations in the water column reflect an oligotrophic character (Klokov et al. Reference Klokov, Kaup, Zierath and Haendel1990). The largest lake in Bunger Hills is Algae Lake, which is part of a 25 km long drainage system that is fed by meltwater streams from the ice sheet and the margin of Apfel Glacier (Fig. 1; Gibson et al. Reference Gibson, Gore and Kaup2002). Meltwater from the ice sheet enters Algae Lake at its eastern end, and via a series of lakes and streams to the south (Fig. 1). To the west, Algae Lake has an outflow across a threshold at c. 10 m a.s.l. into Izvilistaja Inlet and further into Transkriptsii Gulf. At present, the outflow is not permanently active and may vary between years and seasons (Gibson et al. Reference Gibson, Gore and Kaup2002).
Lakes that presently are not fed by meltwater are particularly sensitive to evaporation and precipitation. Amongst these lakes is 8 m lake (unofficial name) in southern Bunger Hills (Fig. 1), whose surface is located at an altitude of 8 m a.s.l. This lake fills an elongated basin with a maximum water depth of 12.5 m that is surrounded by steep slopes with altitudes of c. 40 m. 8 m lake has a restricted catchment area with no surficial outflow. At present, water salinity is higher than in meltwater-fed lakes such as Algae Lake (Melles et al. Reference Melles, Kulbe, Overduin and Verkulich1994b), consistent with observations from other closed lakes in Bunger Hills (Klokov et al. Reference Klokov, Kaup, Zierath and Haendel1990). Instead of direct meltwater input, 8 m lake presently receives influx of concentrated groundwater, which probably provides nutrients that lead to intensive growths of algae mats in the littoral zones of the lake (Melles et al. Reference Melles, Kulbe, Overduin and Verkulich1994b). 17 m lake (unofficial name), in contrast, is a shallow (max. 7.1 m deep) lake on Geographers Island in the northern part of Bunger Hills (Fig. 1), which occupies an enclosed basin at 17 m a.s.l. and is fed by meltwater from adjacent snow banks (Kulbe Reference Kulbe1997).
Freshwater lakes in Bunger Hills contain a benthic fauna and flora consisting of cyanobacteria, chlorophytes, diatoms and mosses (Gibson et al. Reference Gibson, Gore and Kaup2002). The mats are usually less developed in the marginal areas than in deeper parts of the lakes, since lake ice formation leads to abrasion of benthic mats in shallow waters (Gibson et al. Reference Gibson, Gore and Kaup2002, Reference Gibson, Roberts and Van de Vijer2006). In lake basins directly fed by meltwater streams from the ice sheet the high supply of suspended matter leads to turbid water and deposition of mainly siliciclastic sediments. This presently occurs, for example, in the eastern basins of Algae Lake (Gibson et al. Reference Gibson, Gore and Kaup2002).
Methods
Nineteen sediment sequences were previously presented by Melles et al. (Reference Melles, Verkulich and Hermichen1994a, Reference Melles, Kulbe, Overduin and Verkulich1994b, Reference Melles, Kulbe, Verkulich, Pushina, Hubberten and Ricci1997), Kulbe (Reference Kulbe1997), Verkulich et al. (Reference Verkulich, Melles, Hubberten and Pushina2002, Reference Verkulich, Pushina, Sokratova, Melles, Hultsch and Diekmann2007) (Table I). Datasets include lithological descriptions (changes in sediment colour, fabric, grain size and organic remains), total organic carbon (TOC), total nitrogen (N) and total sulfur (S) contents, stable carbon isotope ratios of TOC (δ13C) and mineralogical composition. For two cores (PG1173, PG1180) diatom assemblage data was presented in Kulbe et al. (Reference Kulbe, Melles, Verkulich and Pushina2001) and Verkulich et al. (Reference Verkulich, Pushina, Sokratova, Melles, Hultsch and Diekmann2007). Detailed descriptions of the methods are in the respective publications. For 16 cores 14C-ages of TOC and carbonates have been previously published (Table II). Measurements were made either by Accelerator Mass Spectrometry (AMS) (Lab ID: Ox) or by miniature counter tube (Lab ID: Hv) (Kulbe Reference Kulbe1997, Verkulich et al. Reference Verkulich, Melles, Hubberten and Pushina2002).
Table II. Radiocarbon ages discussed in text. 14C analysis were made either by Accelerator Mass Spectrometry (AMS) (Lab ID: Ox) or by miniature counter tube (Lab ID: Hv). Samples of lacustrine origin (lake) were calibrated with atmospheric calibration (SHcal13, Hogg et al. Reference Hogg, Hua, Blackwell, Buck, Guilderson, Heaton, Niu, Palmer, Reimer, Reimer, Turney and Zimmerman2013), samples of marine orgin (marine) were calibrated with the marine calibration dataset Marine13 (Reimer et al. Reference Reimer, Bard, Bayliss, Beck, Blackwell and Bronk Ramsey2013). A reservoir correction of 1300 a was applied for marine samples (ΔR = 900 a). For calibrated ages minimum (min) and maximum (max.) values of the 95.4% confidence intervals are given. (TOC = total organic carbon), carb. = marine carbonate.
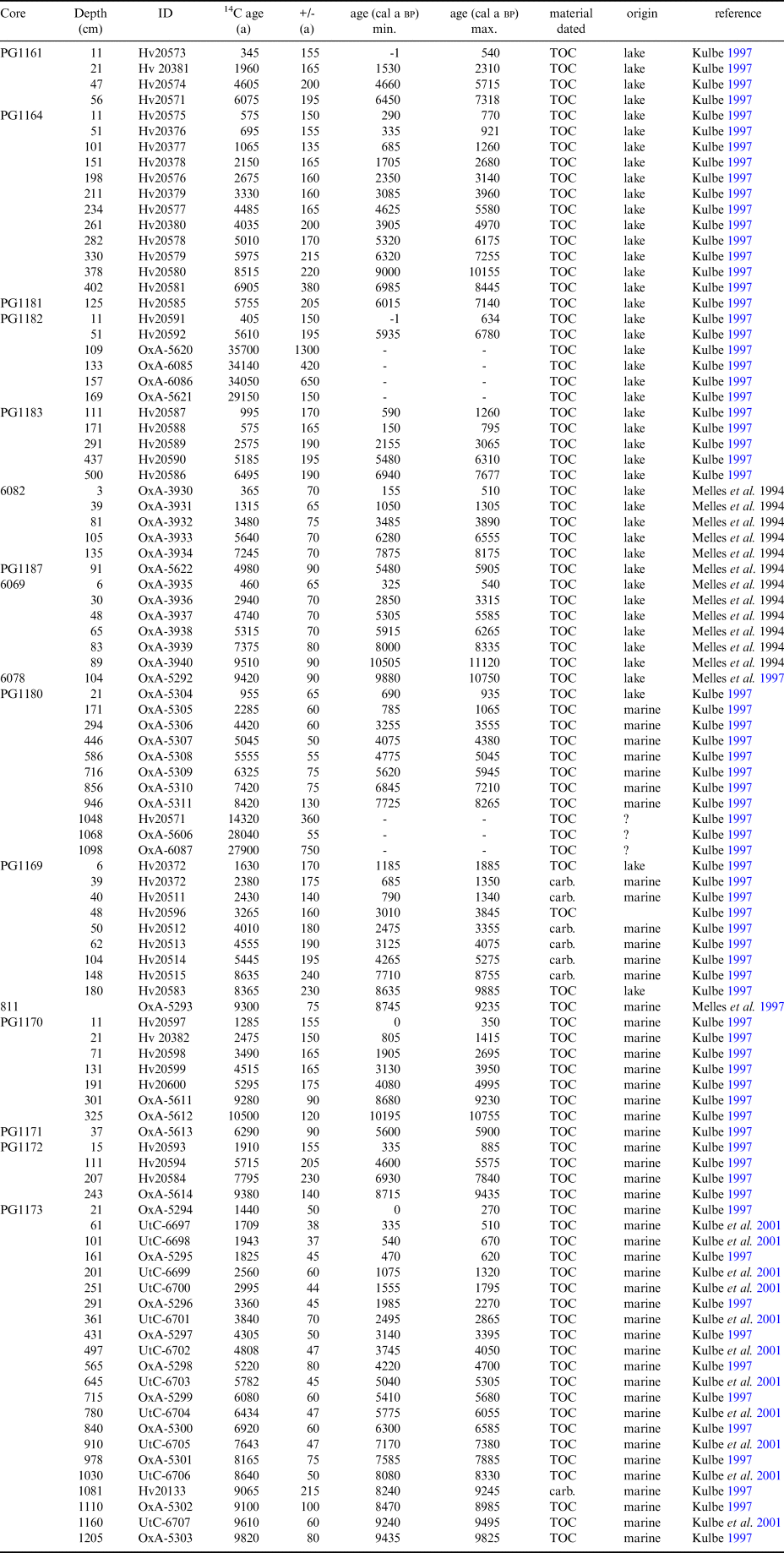
We selected 10 records for which a minimum of four 14C ages are available and obtained age-depth models with the clam software (Blaauw Reference Blaauw2010) by linear interpolation of age tie points or by polynomial regressions. Analyses of freshwater and lacustrine samples were calibrated with atmospheric calibration (SHcal13, Hogg et al. Reference Hogg, Hua, Blackwell, Buck, Guilderson, Heaton, Niu, Palmer, Reimer, Reimer, Turney and Zimmerman2013). The marine calibration dataset Marine13 (Reimer et al. Reference Reimer, Bard, Bayliss, Beck, Blackwell and Bronk Ramsey2013) was used for marine samples, and a reservoir correction of 1300 a (Berkman & Forman Reference Berkman and Forman1996) was applied. Age-depth models for postglacial records deposited under lacustrine conditions were created under the assumption that the radiocarbon ages are not affected by a reservoir effect. This is well justified for modern sedimentation, because i) most lakes in Bunger Hills, which are not in direct contact with a glacier, today have a semi-permanent ice cover (Doran et al. Reference Doran, McKay, Meyer, Andersen, Wharton and Hastings1996) and water columns are well-mixed (Klokov et al. Reference Klokov, Kaup, Zierath and Haendel1990) inhibiting the build up of reservoir ages, and ii) the radiocarbon ages of most near-surface sediment samples are relatively close to modern.
Age-depth models
In core PG1164 from 8 m lake the age model is based on 12 14C ages (Fig. 2). Linear extrapolation through the two most recent dating points provides a 14C age of c. 400 a for the sediment surface and probably reflects disturbance of the sediment-water interface during coring, possibly due to the very high water content of up to 99% in the upper tens of centimetres of sediment (Kulbe Reference Kulbe1997). Age reversals in the sequences PG1164 (Fig. 2) could indicate changes in reservoir ages through time but could also reflect irregular input of reworked older organic carbon (e.g. from the terrestrial catchment). Supply of reworked organic matter, resulting in apparently older sediment ages, is most probable in the highly clastic proglacial sediments, such as those in the lower part of the core 6069 (Fig. 2).

Fig. 2. Lithology and age-depth models for sediment cores from Algae Lake (6069, 6082, PG1183), 8 m lake (PG1164) and 17 m lake (PG1161). Lithology of cores with a few age tie points is also shown (data from Melles et al. Reference Melles, Verkulich and Hermichen1994a, Kulbe Reference Kulbe1997, Verkulich et al. Reference Verkulich, Melles, Hubberten and Pushina2002).
For core PG1161 four 14C ages were obtained (Fig. 2). The sediment properties indicate a hiatus in the record at 21 cm depth due to temporary lake desiccation and associated near-surface sediment freezing. The maximum age of the hiatus is provided by the 14C age of 2.1 ± 0.4 ka bp from the uppermost flaky algal mats at 23 cm depth. The timing of recurrence of algae mat formation, hence the refilling of the lake, was estimated from extrapolation through the modern surface and the only 14C age from the undisturbed algal mat at 11 cm depth.
Age-depth models were obtained for five postglacial records from marine inlets (Fig. 3). For core PG1173, 21 14C analyses (20 on TOC and one on a carbonate bivalve shell, Table II) provide robust age control, with a basal age of sapropel formation of about 9.5 ka bp (Fig. 3). The TOC age from 161 cm depth is apparently too young, possibly reflecting a period of lower reservoir age, e.g. due to inflow of freshwater from melting glacier ice, and thus was excluded from the age model. In the other marine records from Paz Cove (PG 1170 and PG1172) age control is poorly constrained and does not provide evidence for changes in reservoir ages (Fig. 3).

Fig. 3. Age-depth models for sediment cores from Izvilistaja Inlet (PG1180, PG1169) and Paz Cove (PG1170 to PG1173). For core PG 1171 no age model was created due to only one 14C age. Also shown for this core are sulfur (S%), total organic carbon (TOC%) and δ13C of organic carbon.
Estimates of reservoir ages are particularly difficult in epishelf lake settings such as Izvilistaja Inlet (PG1169 and PG1180), where water exchange with the sea is restricted and freshwater inflow is high. In core PG1169, the lower six 14C ages obtained from marine carbonate fossils were corrected for the marine reservoir effect (Fig. 3), while high δ13C values of TOC indicate an origin from freshwater microbial mats (Fig. 4c). 14C ages of TOC from core PG1169 were therefore calibrated with the atmospheric calibration. Employing a polynomial regression, few ages plot slightly to the side of the age-depth curve, however, the data clearly indicate an age at the core base close to 10 ka bp and an altogether continuous and rather constant sedimentation.

Fig. 4. Scatter plots of δ13C vs C/N and TOC data, with symbols referring to sediment cores and colours distinguishing different lithologies within the respective records a. C/N vs δ13C, and b. TOC [%] vs δ13C for t- and p-sediments in all investigated records, c. data for sediment cores from Izvilistaja Inlet, d. data for sediment cores from Paz Cove, e. data for sediment cores from Algae Lake, and f. data for sediment cores from 8 m and 17 m lake (all data from Kulbe Reference Kulbe1997, see Fig. 1 for coring locations).
In core PG1180, seven 14C ages from the marine sapropelic sediments and one 14C age from the freshwater part of the record were available (Fig. 3). The uppermost sample in the marine sediments was excluded from the age-depth model, which is based on linear interpolation between data points, since this part of the record was probably deposited under the increasing influence of freshwater and thus the marine reservoir estimate of 1300 a is probably not fully applicable there.
At two sites (PG1180, PG1182), 14C ages were obtained from till deposits (Figs 2 & 3). The ages are 28.0 ± 0.05 and 27.9 ± 0.8 ka bp in core PG1180 and 29.2 ± 0.7 to 35.0 ± 1.3 ka bp in core PG1182. Given the subglacial deposition, the organic carbon in the tills must have been reworked from older deposits. Due to the potentially mixed sources of carbon the ages were neither corrected for a reservoir effect nor calibrated.
Depositional environments
Identification of former depositional environments from sediment cores is crucial for the discussion of RSL changes (lacustrine vs marine depositional environments), shifts in ice sheet margins and glacier extent (subglacial vs proglacial vs open marine/limnic environments) and reconstruction of past climatic conditions (interpretation as proxy data). In this section we review published datasets (TOC, δ13C values, lithology, mineralogy, diatom assemblage) of 19 sediment records in order to characterise different depositional environments and to derive possible interpretations of the data with respect to climate conditions.
Subglacial deposits
Cores PG1161, PG1164, PG1170, PG1171, PG1172, PG1173, PG1180, PG1182, PG1183, PG1184, PG1185 and PG1186 contain highly consolidated, diamictic sediments at their base, interpreted as a till (Kulbe Reference Kulbe1997). TOC contents of the tills are generally low (< 0.02%, Fig. 4b). At sites PG1180 and PG1182, however, they reach 0.2 to 1.3%, indicating incorporation of reworked organic matter (Fig. 4b). δ13C values of organic matter (OM) are mostly lower than -21‰, reaching values as low as -29.9‰ at site PG1182 (Fig. 2a), which is in the range of organic matter (OM) of terrestrial and freshwater origin (e.g. Bramley-Alves et al. Reference Bramley-Alves, Wankek, French and Robinson2015, Yangyang et al. Reference Yangyang, Jing, Yaguang, Xin, Libin, Pingqing, Xiaodong and Emslie2016 and references therein). Depleted values are found at sites that are terrestrial at present (e.g. sites PG1164, PG1182), but also at site PG1171, which is marine. At sites PG1173 and PG1180 δ13C values of OM in tills (-22.2 ± 1.1‰) overlap within 2σ with the range of Holocene marine samples at site PG1173 (20.0 ± 0.7‰) and site PG1180 (19.8 ± 0.8‰) (Fig. 4c & d). This could indicate marine contributions to OM in the till. However, tills at both sites are free of halite (NaCl; Table III), which instead suggests a terrestrial/freshwater formation.
Table III. Samples analysed for the presence (x)/absence (-) of halite in five sediment cores from Bunger Hills (data from Kulbe Reference Kulbe1997).

Proglacial deposits
All cores contain a clastic-rich sediment unit that mostly consists of clayey to sandy silts of olive to brownish colours and either overlies the consolidated, diamictic unit or forms the lowermost unit cored (Kulbe Reference Kulbe1997). At some locations, these sediments consist of laminated clays or contain gravel. TOC contents mostly are < 0.5%, but at sites PG1172 and PG1185 reach up to 2% (Fig. 4b). The clastic-rich sediments were probably deposited in a proglacial environment, where input of siliciclastic matter dominated and deposition of OM was strongly restricted due to dilution with siliciclastic components or low contemporaneous productivity. Proglacial sedimentation presently occurs at sites PG1184 and PG1186 in eastern Algae Lake, where meltwater streams from the ice sheet supply large amounts of suspended matter (Gibson et al. Reference Gibson, Gore and Kaup2002). Consequently, modern sediments of cores PG1184 and PG1186 consist of laminated clayey silts with very low TOC contents.
OM in the proglacial deposits could be autochthonous, originating from phytoplankton or benthic organisms. Alternatively, it could be reworked from older strata, namely from till deposits, which become exposed and potentially reworked during successive ice retreat. A reworked source is indicated for sites PG1183, PG1164, and PG1171, where the tills and the overlying proglacial sediments have very similar C/N and δ13C values (Figs 4d–f). At sites PG1180 and PG1182, in contrast, δ13C values and C/N ratios of the proglacial deposits differ from the underlying till deposits, suggesting a contemporaneous origin (Fig. 4c & e).
Due to the glaciological setting of Bunger Hills, the depositional environment (marine or lacustrine) of the proglacial sediments can give important information on grounding line positions or changes in RSL. At coring location PG1173 in Paz Cove, δ13C values from the proglacial sediments (1245 to 1211 cm core depth) are within the range of values for the biogenic marine sediments above (-21.7 ± 0.9‰ vs 20.0 ± 0.7‰, Fig. 4d). In addition to the occurrence of marine salts (1225 cm depth, Table III) and marine diatoms (Verkulich et al. Reference Verkulich, Pushina, Sokratova, Melles, Hultsch and Diekmann2007) this indicates a marine environment for deposition. In core PG1180 from Izvilistaja Inlet clastic-rich proglacial sediments (1008 to 946 cm core depth) are laminated and have δ13C values ranging from -25.5 to -23.6‰ (Fig. 4a). The latter is typical for terrestrial mosses, lichen, algae and lacustrine mosses in Antarctica (e.g. Yangyang et al. Reference Yangyang, Jing, Yaguang, Xin, Libin, Pingqing, Xiaodong and Emslie2016 and references therein) and thus points to a freshwater or terrestrial origin of the OM. However, the OM in these sediments probably was reworked into a marine environment, as suggested by enrichment in sulfur (up to 4%) and the presence of marine salts (at 978 cm, Table III) and marine diatoms above 976 cm core depth (Verkulich et al. Reference Verkulich, Pushina, Sokratova, Melles, Hultsch and Diekmann2007). The autochthonous production may have been relatively low due to high suspended sediment load in the water column. The terrestrial or lacustrine OM could originate from tills with their respective TOC contents. However, an OM transport from a lake into the marine realm cannot be excluded. A modern example for such a transport is Algae Lake, where benthic microbial mats lift off the lake floor, float to the downwind (western) end of the lake and further via Algae River into Izvilistaja Inlet (Gibson et al. Reference Gibson, Gore and Kaup2002).
In Algae Lake, at sites PG1181, PG1184 and PG1185, δ13C values in the proglacial sediments are more depleted (< -22‰) than in the overlying biogenic sediments (Fig. 4e). This probably reflects a reduced photosynthetic activity of benthic microbial mats and mosses when clastic input is high and light availability is reduced by suspension. In addition, high sediment input leads to high sedimentation rates and potentially unstable lake floor sediments, which may limit the establishment of benthic communities (e.g. Priddle & Heywood Reference Priddle and Heywood1980). The OM in the proglacial sediments could therefore also contain higher proportions of reworked material, e.g. from terrestrial biomass, that forms in and around meltwater streams and depleted δ13C values could be a signal for contribution of allochthonous OM.
Biogenic freshwater deposits
Highly biogenic sediments were recovered in the upper core parts at all freshwater lake sites, except for the sites PG1184 and PG1186 proximal to the ice sheet margin, where proglacial sediments extend to the benthic sediment surface. Biogenic sediments are composed of layers of mosses and microbial mats (Fig. 2). TOC contents range from 1.5 to 14%. The high variability within the records may reflect changes in benthic productivity, in terrestrial input and in the preservation of the organic matter. It may also be due to a patchy distribution of the mosses and microbial mats on the lake floors (Kudoh et al. Reference Kudoh, Tsuchiya, Aykawa, Imura and Kanda2003).
OM from the lacustrine sediments shows a range of -4.6 to -29.1‰ in δ13C values (Fig. 2c, e, f). Generally, high (less depleted) δ13C values point to OM produced under CO2 limited conditions, either due to high photosynthesis rates, which occur for example in moats, or due to limited CO2 diffusion as it occurs in shallow waters and glacial cryoconite holes (Doran et al. Reference Doran, Wharton, Des Marais and McKay1998). δ13C values > -15‰ were found in freshwater algae and microbial mats in shallow water depths (< 2 m) in Antarctic lakes (e.g. Yangyang et al. Reference Yangyang, Jing, Yaguang, Xin, Libin, Pingqing, Xiaodong and Emslie2016) and in the perennially ice-covered White Smoke Lake, a freshwater epishelf lake in Bunger Hills (Fig. 1, Doran et al. Reference Doran, Wharton, Lyons, Des Marais and Andersen2000). We therefore suggest that organic matter with δ13C values > -15‰ is of freshwater origin, probably derived from microbial mats and algae growing in shallow waters. In deeper waters, where productivity is low and water is saturated with CO2, fractionation is much higher, resulting in lower (more depleted) δ13C values (> -30‰; Doran et al. Reference Doran, Wharton, Des Marais and McKay1998).
In core PG1164 from 8 m lake low δ13C values (< -24‰) in the biogenic sediments correspond to higher C/N ratios (> 10; Fig. 4f). This probably reflects the higher proportions of subaquatic mosses relative to microbial mats from the shallow marginal areas of the lake. High C/N ratios (18.9) have been reported for subaquatic mosses in oligotrophic lakes from East Antarctica (Kudoh et al. Reference Kudoh, Tsuchiya, Aykawa, Imura and Kanda2003), which could indicate mosses in sediments.
In 17 m lake on Geographers Island in north-western Bunger Hills, flaky, dried microbial mats occur between 56 and 21 cm depth in core PG1161 (Fig. 2). These sediments indicate that the lake desiccated in the past or was shallow enough to allow winter freezing of the entire water column and further into the underlying sediments (Melles et al. Reference Melles, Kulbe, Overduin and Verkulich1994b). Water-rich, undisturbed microbial mats in the upper 21 cm of the core indicate re-filling of the lake. Lower δ13C values of the OM in 17 m lake than in 8 m lake probably are due to a higher proportion of microbial mats, reflecting the shallower water depths of the lake. At present, 17 m lake is less saline than 8 m lake (Melles et al. Reference Melles, Kulbe, Overduin and Verkulich1994b). This may reflect a recent infilling with meltwater from permanent snowbanks in the catchment (Adamson & Colhoun Reference Adamson and Colhoun1992). The finding of a desiccation event in the sediment record of 17 m lake, however, suggests that salinities may have changed considerably during the lake’s history.
Marine deposits
In the marine inlets, proglacial sediments are overlain in most cases by sandy silts (Fig. 3), which are mostly massive and have TOC contents from 0.5 to 3.5% in PG1169, PG1170, PG1172 (Fig. 5) and PG1171 (Fig. 3). The lack of stratification may indicate intense bioturbation. At two sites (PG1173, PG1180), the proglacial sediments are overlain by sapropels, which reflect deposition in anoxic bottom waters. They differ from other marine sediments by having generally higher TOC contents (1.5 to 8%), high water contents (c. 75%, Kulbe Reference Kulbe1997) as well as a high concentration of diatoms (Kulbe et al. Reference Kulbe, Melles, Verkulich and Pushina2001, Verkulich et al. Reference Verkulich, Pushina, Sokratova, Melles, Hultsch and Diekmann2007).

Fig. 5. Proxies shown vs age for records from marine inlets a. Izvilistaja Inlet and b. Paz Cove (data from Kulbe Reference Kulbe1997).
In the marine sapropels of core PG1173 from Paz Cove and Izvilistaja Inlet (PG1180) the most abundant diatom frustules are from Chaetoceros (mainly resting spores, rs), which comprise 35 to 97% (mean 77.7 ± 10.8%) of the diatom valves in core PG1173 (Fig. 6). In coastal Antarctic environments, intensive formation of Chaetoceros resting spores occurs in the upper layer of a well-stratified water column, either from intensive sea ice melt in spring or supply of meltwater from the continent (e.g. Cremer et al. Reference Cremer, Gore, Melles and Roberts2003, Leventer et al. Reference Leventer, Domack, Dunbar, Pike, Stickley, Maddison, Brachfeld, Manley and McClennen2006). Chaetoceros blooms are supported by iron input from dust or from meltwater, as that supplied to calving bays during deglaciation (e.g. Leventer et al. Reference Leventer, Domack, Dunbar, Pike, Stickley, Maddison, Brachfeld, Manley and McClennen2006). In the inlets of Bunger Hills, Chaetoceros blooms are probably intensified, when nutrient input from land is high, e.g. during periods of high run-off of clastic-rich meltwater, and are probably less intensive when sea ice is more persistent or freshening of the water column exceeds the salinity tolerance of Chaetoceros spp. Sea ice-related species (Entimoneis kjellmannii, Fragilariopsis curta, F. cylindrus, F. obliquecostata, F. ritscheri, F. sublinearis, Porosira glacilis, Navicula directa) comprise 4.0 ± 3.3% in PG1173 (Verkulich et al. Reference Verkulich, Pushina, Sokratova, Melles, Hultsch and Diekmann2007) and 2.7 ± 1.8% in PG1180 (Verkulich et al. Reference Verkulich, Pushina, Sokratova, Melles, Hultsch and Diekmann2007), which is much less than found in other marine inlets from the East Antarctic coast (e.g. Cremer et al. Reference Cremer, Gore, Melles and Roberts2003). In Izvilistaja Inlet (PG1180) Cocconeis pinnata, which is a brackish/benthic species (Roberts & McMinn Reference Roberts and McMinn1998), is abundant in higher proportion than in Paz Cove (PG1173), probably reflecting higher proportions of freshwater, e.g. from Algae Lake or Edisto Glacier. In Paz Cove (PG1173), the presence of F. kerguelensis in the lower part of the sediment sequence, points to connection to the open ocean.

Fig. 6. Selection of diatoms identified in cores PG1173 and PG1180. Chaetoceros resting spores (Chaet. rs.). Species grouped as sea ice diatoms (SI) are Entomoneis kjellmannii, Fragilariopsis curta, F. cylindrus, F. obliquecostata, F. ritscheri, F. sublinearis, Porosira glacialis, Navicula directa. F. curta gr. comprises Fragilariopsis curta and F. cylindrus. Cocconeis pinnata is a fresh/brackish species and Fragilariopsis kerguelensis (F. kerguel.). Freshwater (FW) are dominated by Amphora veneta (data from Kulbe et al. Reference Kulbe, Melles, Verkulich and Pushina2001, Verkulich et al. Reference Verkulich, Pushina, Sokratova, Melles, Hultsch and Diekmann2007).
The δ13C values of organic carbon show a low variability within and between the marine records compared to the range found in the lacustrine records (Figs 4d & 5). The mean δ13C values in the sapropels (-19.9 ± 0.7‰ in PG1173 and -19.8 ± 0.8‰ in PG1180) are similar to those found at sites with more clastic-dominated sedimentation (-20.0 ± 0.7‰ in PG1170, -20.2 ± 0.5‰ in PG1171 and -20.6 ± 0.6‰ in PG1172). The values are comparable to δ13C values from sapropels in coastal marine inlets in other East Antarctic oases (-22 to -18‰, Kirkup et al. Reference Kirkup, Melles and Gore2002, Berg et al. Reference Berg, Leng, Kendrick, Cremer and Wagner2013). Gradual trends in δ13C values as well as the sharp decrease in δ13C around 2.2 ka bp in both cores is not clearly reflected in the diatom record. In particular, δ13C values are not associated with peaks in sea ice diatoms or maxima/minima in Chaetoceros resting spores (Figs 5 & 6). This suggests that the δ13C values are not directly controlled by the proportion of sea ice diatoms on biomass and therefore cannot simply be interpreted as an indicator of sea-ice coverage. Changes in δ13C more probably reflect a signal of phytoplankton productivity, carbon utilisation and carbon source composition.
In Izvilistaja Inlet a transition from marine to freshwater conditions is reflected in core PG1180 from the inner part of Izvilistaja Inlet, by a lithological change from marine sapropels to microbial mats at 131 cm core depth. The transition is accompanied by a shift to higher δ13C values and enrichment in sulfur (Fig. 5a). The occurrence of mosses above 96 cm and the establishment of a freshwater diatom assemblage show that no marine incursions occurred afterwards (Fig. 6, Verkulich et al. Reference Verkulich, Pushina, Sokratova, Melles, Hultsch and Diekmann2007). In core PG1169, from the outer part of Izvilistaja Inlet, marine fossils point to deposition under marine conditions (Kulbe Reference Kulbe1997, Verkulich et al. Reference Verkulich, Pushina, Sokratova, Melles, Hultsch and Diekmann2007). The upper c. 6 cm of that core are composed of freshwater microbial mats, reflecting the present-day freshwater infill of the inlet. High δ13C values throughout the record (-16.1 ± 4.4‰; Fig. 5a) suggest that OM mainly is of freshwater rather than marine origin. In the marine sediments, this can be explained by microbial mat supply from Algae Lake. Modern day microbial mats in Algae Lake partly float and drift towards the downwind outlet of the lake, with the dominant wind direction (Gibson et al. Reference Gibson, Gore and Kaup2002). The mats may further become transported into Izvilistaja Inlet, when water flows through Algae River. An allochthonous origin of at least parts of the OM in core PG1169 is supported by the high TOC contents (1.9 ± 0.8%) in the sandy sediments of this relatively shallow site (11.1 m water depth).
Depositional histories
Algae Lake
At sites PG1184, PG1185, PG1186, PG1183 and PG1182, till was recovered at the core bases, pointing to inundation of the basin that is presently occupied by Algae Lake by a grounded glacier or ice sheet. 14C ages of 29.2 ± 0.7 to 35.0 ± 1.3 ka bp in the till of core PG1182 provide important information concerning maximum till age, with the latest corresponding with Marine Isotope Stage (MIS) 3. Furthermore, organic matter in the tills and their ages suggest ice-free areas with biogenic accumulation in the catchments of the coring sites some time prior to the Last Glacial Maximum.
Proglacial sediments overlying the till in Algae Lake indicate a period of glaci-lacustrine sedimentation before biogenic sedimentation started in the basins distal to the present-day ice sheet margin (PG1183, 6082, 6069, 6078) around 9.8 to 7.3 ka bp (Fig. 7a). At coring locations PG1181 and PG1182 clastic-dominated sedimentation remained high even until 6.6 ± 0.5 ka bp (Fig. 2). These coring locations are close to streams that presently drain lakes fed from snowbanks (PG1182) and indirectly from the ice sheet via Lake Dalekoje (PG1181). A later onset of biogenic sedimentation at these sites probably indicates that clastic input remained high and unstable lake floor sediments and high suspension load hampered establishment of benthic communities longer than in other parts of Algae Lake.

Fig. 7. Proxies shown vs age for records from lakes a. Algae Lake, and b. 8 m lake (PG1164) and 17 m lake (PG1161) (data from Kulbe Reference Kulbe1997 and Verkulich et al. Reference Verkulich, Melles, Hubberten and Pushina2002).
In core PG1183 low δ13C values and high C/N ratios in the lower biogenic deposits (Figs 2 & 7) indicate high proportions of mosses in the initial sediments formed after proglacial conditions ceased. This interpretation is consistent with high moss abundances described for the lowermost biogenic sediments in cores from sites 6078, 6082 and 6069 (Fig. 2; Verkulich et al. Reference Verkulich, Melles, Hubberten and Pushina2002). In Antarctica, perennial mosses colonize lake bottoms with oligotrophic conditions and low light intensities (Priddle & Heywood Reference Priddle and Heywood1980). Oligotrophic conditions in Algae Lake during early Holocene times are suggested by very low diatom abundances in the respective sediments (Verkulich et al. Reference Verkulich, Melles, Hubberten and Pushina2002). Another common pattern in the records PG1183, 6082, 6069 and 6078 is enrichment in S from the onset of biogenic sedimentation until 5.7 ± 0.2 ka bp (Fig. 7a). High S contents probably result from oxygen depletion during degradation of mosses and microbial mats. Another source of S could be the mineral thenardite (Na2SO4), which precipitates in littoral areas of lakes in Bunger Hills during lake ice formation and accumulates in deeper parts of the lakes (Klokov et al. Reference Klokov, Kaup, Zierath and Haendel1990). Enrichment in S may point to a more persistent lake-ice cover during that time.
A decrease in TOC values at 5.7 ± 0.2 ka bp (Fig. 7a) could indicate lower productivity but also less efficient preservation of OM in the lake. The latter is more probable, since a contemporaneous increase in δ13C values of OM instead point to an increase in productivity or to an increase in the proportion of algal mats from the shallow littoral areas of the lake, which is consistent with less lake ice and better mixing of the water column. The observed decrease in mosses relative to microbial mats at 5.7 ± 0.2 ka bp is supported by lower C/N ratios (Fig. 7a). The subsequently low variability of δ13C values points to a period of relative stability with respect to nutrient supply and productivity. Flourishing benthic communities in the lake at that time are also indicated by increasing abundance of benthic diatoms in cores 6078, 6082 and 6069 (Verkulich et al. Reference Verkulich, Melles, Hubberten and Pushina2002).
At 1.3 ± 0.3 ka bp, TOC values decrease and a drop in δ13C values indicates a short-term decrease in productivity. Maximum TOC values from 0.5 to 0.2 ka bp probably reflect recurring high productivity, however, δ13C values are highly variable compared with the time before 1.3 ka bp, pointing to less stable conditions in the lake.
Other lakes
In contrast with Algae Lake, 8 m and 17 m lake today are not fed by meltwater run-off from the ice sheet or glacier margins. Sedimentary records from these lakes, therefore, may reflect changes in temperature and precipitation more sensitively than records from Algae Lake.
In 17 m lake (PG1161), till is overlain by highly clastic sediments that show a fining upward trend from sand to sandy silt and are topped by a gravel layer at 70 cm depth. The clastic sediments were probably deposited during ice retreat, while the gravel layer may reflect a lag deposit indicative of sub-aerial exposure of the lake bottom or winnowing of sediment by water, such as by a meltwater stream (e.g. Gibson et al. Reference Gibson, Gore and Kaup2002). Above the gravel, sandy-silty sediments with TOC contents of 0.2 to 0.4% point to lacustrine sedimentation at the site. Around 6.9 ± 0.4 ka bp microbial mats colonized the lake (Figs 2 & 7b), indicating lacustrine fresh conditions.
In 8 m lake (PG1164), biogenic lake sedimentation started around 7.8 ± 0.7 ka bp (Figs 2 & 7b). Increasing TOC values from 1.5 to 8%, high C/N ratios and low δ13C values until 5.9 ± 0.2 ka bp indicate successive colonisation of the lake bottom by aquatic mosses under oligotrophic conditions. A distinct peak in C/N ratios around 6 ka bp could indicate in-wash of degraded OM from the catchment, which could also explain the lower TOC values during this interval by dilution with clastic matter. From 5.7 ± 0.2 to 2.6 ± 0.3 ka bp, C/N and δ13C values show lower variability than in the previous interval, pointing to more stable conditions in the catchment or within the lake, particularly with respect to nutrient supply and terrestrial input.
After 2.6 ± 0.3 ka bp, higher δ13C values in 17 m lake (Fig. 7b) probably reflect more saline conditions due to successive shallowing, culminating in desiccation of the lake after 2.1 ± 0.4 ka bp (Fig. 7a). At the same time, C/N values in the record from 8 m lake shift from > 14 to < 10 and δ13C values increase from < -24 to > -24‰. This reflects a shift from higher proportions of mosses to higher proportions of microbial mats (Fig. 7b). Productivity of microbial mats in the littoral areas of the lake is enhanced when nutrient supply from surficial and sub-surface runoff is high (Melles et al. Reference Melles, Kulbe, Overduin and Verkulich1994b) and lake ice coverage is thinner. The shift could therefore reflect a period of less lake ice and higher nutrient input from land. Alternatively, the increase in microbial mats could be due to an increase in salinity, which results in less favourable conditions for freshwater water mosses (Kudoh et al. Reference Kudoh, Tsuchiya, Aykawa, Imura and Kanda2003).
The period of increased microbial mat deposition in 8 m lake was interrupted from 1.6 ± 0.3 to 1.1 ± 0.3 ka bp, which could either reflect dilution or an increase in lake ice and a decrease in nutrient input. The latter is supported by the hiatus in the record from 17 m lake (Figs 2 & 7b), which falls within this interval and most probably was connected to colder and drier conditions. After 1.1 ± 0.3 ka bp high δ13C values and maximum TOC contents in 8 m lake indicate recurrence of high productivity of microbial mats, probably coincident with the refilling of 17 m lake c. 0.6 ± 0.4 ka bp.
Marine inlets
Till at the base of cores PG1180, PG1170, PG1171, PG1172 and PG1173 (Fig. 3) point to past glacier or ice sheet coverage and grounding in Paz Cove and Izvilistaja Inlet after c. 28.0 ka (Table II). In core PG1180 from Izvilistaja Inlet, an age of 14.3 ± 0.3 14C ka in postglacial sediments could be interpreted as a minimum age of the ice sheet or glacier retreat at this site. However, this age could also be erroneously old, due to admixture of reworked, older organic matter, which is entrained in the underlying till.
Proglacial sediments overlying the tills, coupled with the presence of halite and/or marine diatoms, and high S concentrations, indicate glaci-marine sedimentation at sites PG1171, PG1173 and PG1180. At site PG1172 high δ13C values of -16.3‰ V-PDB indicate incorporation of OM derived from freshwater mosses or microbial mats (Fig. 6), however, this OM may not necessarily be autochthonous, since marine diatoms were identified in the sediments (Verkulich et al. Reference Verkulich, Pushina, Sokratova, Melles, Hultsch and Diekmann2007). In cores PG1170 and PG1172 from Paz Cove, 14C ages of 10.4 ± 0.3 ka bp and 9.1 ± 0.3 ka bp were obtained from clastic sediments (clayey to sandy silt and clays to silty sands) above the till (Fig. 3) providing a minimum age of ice retreat from these sites.
Biogenic sedimentation of diatomaceous sapropels started at 9.6 ± 0.2 ka bp at site PG1173 (Fig. 3). Around the same time (8.9 ± 0.5 ka bp), sedimentation became more fine-grained and organic rich at site PG1170 (Fig. 5b). At the more coastal coring locations in western Paz Cove, clastic-dominated sedimentation lasted longer, terminating around 7.3 ± 0.4 ka bp (PG1172) and 5.7 ± 0.2 ka bp (PG1171; Fig. 3). This may reflect a successive ice retreat to the west of Paz Cove. An opposite picture is provided by Izvilistaja Inlet, where marine sedimentation started at 9.4 ± 0.9 ka bp in the outer, western part of the inlet (PG1169), while the inner basin was subjected to high clastic input until 7.9 ± 0.1 ka bp (PG1180) (Fig. 5a). However, this does not necessarily reflect ice retreat to the east, it could also be due to a particularly high clastic sediment supply from Algae Lake via Algae River during the early Holocene, possibly reflecting enhanced meltwater supply.
High productivity at site PG1173 in Paz Cove occurred from the onset of sapropel deposition at 9.6 ± 0.2 until 7.9 ± 0.1 ka bp. High Chaetoceros rs. concentrations probably reflect high nutrient supply from meltwater input from land (Fig. 6). During the beginning of this period, until c. 8.5 ka bp, sea ice diatoms reached a maximum abundance (Fig. 6).
At 7.9 ± 0.1 ka bp a reduction in clastic input led to the onset of marine sapropel deposition in Izvilistaja Inlet (PG1180). The decrease in Chaetoceros blooms in Paz Cove at the same time could be due to reduced nutrient input, increased water column mixing and/or freshening, which reduces the photosynthetic efficiency of Chaetoceros sp. (Petrou & Ralph Reference Petrou and Ralph2011). A relatively high proportion of the brackish C. pinnata in Izvilistaja Inlet (PG1180) from 7.9 ± 0.1 ka bp to c. 5.7 ± 0.3 ka bp point to reduced water exchange with the ocean and could be an effect of RSL lowering or thickening of Edisto Glacier. More restricted exchange with the ocean is also indicated by a reduction in open ocean F. kerguelensis in Paz Cove (PG1173) after 7.0 ± 0.1 ka bp. This was accompanied by lower productivity in both inlets as indicated by lower TOC and S contents and relatively low δ13C values throughout this period (Figs 5 & 9). From 5.7 ± 0.3 to 2.2 ± 0.1 ka bp, higher abundances of Chaetoceros rs. (generally > 78%), increasing TOC contents and less depleted δ13C values indicate high productivity in both marine inlets (Figs 5 & 6). The trend was interrupted by a short period from 4.5 to 4 ka bp when nutrient supply was lower and conditions were less favourable for Chaetoceros blooms. The most prominent shift in the marine δ13C records occurs at 2.2 ± 0.1 ka bp, when δ13C values decrease (PG1180, PG1173, PG1170), and TOC contents decrease in some records from Paz Cove (PG1173, PG1170) (Fig. 5). The shift in the δ13C record is accompanied by a decrease in Chaetoceros rs., but not so much in sea ice diatoms, which points to a change in water column properties, probably related to changes in stratification/mixing or salinity rather than an increase in sea ice (Cremer et al. Reference Cremer, Gore, Melles and Roberts2003). The decrease in δ13C values could reflect reduced phytoplankton productivity or change in phytoplankton assemblage, which could be induced by freshening of the water column. In PG1169 (outer Izvilistaja Inlet) δ13C values continuously increase after c. 2.2 ka bp, which points to an increase in allochthonous OM deposition of freshwater microbial mats, probably from Algae Lake and could be an indication for successive reduction in marine water contribution to Izvilistaja Inlet. In inner Izvilistaja Inlet (PG1180), increasing TOC contents probably reflect increasing preservation of organic matter due to increasingly stable water column stratification since c. 2.2 ka bp. The site became permanently fresh at 1.4 ± 0.1 ka bp (Figs 5 & 9). At site PG1169, further to the west, freshwater microbial mats occur later (0.25 + 0.2/-0.1 ka bp), which probably reflects the onset of a permanent freshwater layer in the adjacent Transkriptsii Gulf.
Environmental history
Ice sheet and glacier fluctuations
Tills at the base of the marine and lacustrine sediment sequences suggest ice sheet or glacier ice coverage of the core sites during the last glacial advance in Bunger Hills. This is consistent with OSL dating of hillside glaciofluvial sediments, which indicate that nunataks emerged from the downwasting ice sheet and most hilltops were completely exposed by c. 20 ka bp, while sites at lower altitudes, like the Algae Lake trough, remained ice-filled (Gore et al. Reference Gore, Rhodes, Augustinus, Leishman, Colhoun and Rees-Jones2001). Ages of c. 35 to 28 14C ka, obtained from organic carbon entrained in the tills at coring sites PG1180 and PG1182 are probably from reworked terrestrial deposits and probably support the timing of late Pleistocene exposure of Bunger Hills.
In south-western Bunger Hills deglaciation was associated with the formation of ice-dammed glacial lakes, which are indicated by terraces at altitudes of c. 20 m a.s.l. (Colhoun & Adamson Reference Colhoun and Adamson1989). The largest and highest glacial lake (Dolgoe Lake; Fig. 1) was dammed by ice lying in the present-day Algae Lake basin. OSL ages from glacial lakeshore sediments indicate that it had drained substantially by 15 ka bp and attained its present lake level after 12.4 ka bp (Gore et al. Reference Gore, Rhodes, Augustinus, Leishman, Colhoun and Rees-Jones2001). The timing of initial deglaciation and the duration of proglacial siliciclastic deposition cannot be well constrained from the lacustrine and marine sediment records investigated in this study due to a lack of age control on these strata. The records are, however, consistent with a formation that post-dates drainage of the glacial lakes. Further evidence for the onset of deposition during progressive deglaciation comes from Izvilistaja Inlet (PG1180) and Paz Cove (PG1173), where laminated, clastic-rich sediments were deposited under marine conditions, before highly biogenic sedimentation commenced at 7.9 ± 0.3 ka bp and 9.6 ± 0.2 ka bp, respectively. The presence of marine water in the bays of Bunger Hills during that time suggests that at least parts of Shackleton Ice Shelf and/or glaciers surrounding the hills were not completely grounded, allowing marine waters to fill the depressions.
Clastic input into eastern and central Paz Cove decreased around 9.6 ± 0.2 ka bp (PG1173, PG1170), but remained high in western Paz Cove (PG1172, PG1171), Algae Lake (PG1183, 6069, 6078, 6082) as well as in adjacent Izvilistaja Inlet (PG1180) and in the lakes upstream in the drainage system (e.g. Burevestnik Lake; Bolshiyanov et al. Reference Bolshiyanov, Verkulich, Klokov and Arslanov1991) (Fig. 8a). This indicates that ice sheet margins had retreated to a more distal position (relative to the coring locations) in Cacapon Inlet as also suggested by sediment burial ages (Gore et al. Reference Gore, Rhodes, Augustinus, Leishman, Colhoun and Rees-Jones2001) and 14C ages of mumiyo deposits, which indicate snow petrel colonisation of southern Bunger Hills since c. 10.1–11.5 ka bp (Verkulich & Hiller Reference Verkulich and Hiller1994). The flow direction of water from Algae Lake into Izvilistaja Inlet is confirmed by the presence of marine conditions in Izvilistaja Inlet, with no clear indication of a marine ingression found in the proglacial sediments from Algae Lake (PG1182, Fig. 8a). Input of clastic matter via Algae River is also supported by the virtually synchronous onset of biogenic sedimentation in Algae Lake (PG1183) and of marine sapropels in Izvilistaja Inlet (PG1180) around 7.5 ± 0.4 and 7.9 ± 0.3 ka bp, respectively. While large-scale ice retreat ceased around 8.0 ± 0.3 ka bp, melting of remnant local ice masses probably continued in southern and northern Bunger Hills until 6.6 ± 0.5 ka (Fig. 8b). The latter is indicated by a delayed onset of biogenic sedimentation at coring locations PG1181 and PG1182 in Algae Lake, higher input of reworked organic matter into 8 m lake until 5.9 ± 0.2 ka bp and freshwater influence in western Paz Cove, indicated by low δ13C values in proglacial clastic sediments in cores PG1172 (9.0 ± 0.3 ka to 7.3 ± 0.5 ka bp) and PG1171 (prior to 5.7 ± 0.2 ka bp) (Fig. 9).

Fig. 8. Reconstruction of glacier fluctuations and clastic matter input into lakes and marine inlets. Time slices are also distinguished in Fig. 9.

Fig. 9. Paleoclimatic interpretation of marine and lacustrine records from Bunger Hills. Periods underlain in blue were probably cooler than periods underlain in orange colours. Some disagreement between records probably arises from uncertainties in the age models of each of the records. Horizontal dotted lines indicate correlation with the record from Pol'anskogo Lake (core 811, Melles et al. Reference Melles, Verkulich and Hermichen1994a) and are interpreted as periods of advanced grounding line of Apfel Glacier and expanded Edisto Glacier. SI = sea ice diatom species, FW = freshwater diatom species. Sea ice diatoms and Chaetoceros rs. for core PG1173 are shown as 10 point running average.
Two sets of moraines flanking the present-day extent of Edisto Glacier were ascribed to two to three Holocene glacier advances (Adamson & Colhoun Reference Adamson and Colhoun1992, Augustinus Reference Augustinus2002). Grounding-line fluctuations of Apfel Glacier during the Holocene are also consistent with the diatom record from core 811 from epishelf Pol’anskogo Lake, which reflects two to three periods of freshening (Figs 7 & 8, Melles et al. Reference Melles, Kulbe, Verkulich, Pushina, Hubberten and Ricci1997) that may be associated with a restriction of marine water intrusion due to glacier advance (Fig. 8b & d). 14C age assignment for the medial moraine was obtained from a reworked shell that gives a maximum age of the advance of 7.0 ± 0.2 ka bp (Adamson & Colhoun Reference Adamson and Colhoun1992). The only radiocarbon age from the base of core 811 (Table I) does not provide reliable ages for the freshening events, but constrains their ages to be younger than 9.0 ka bp (Melles et al. Reference Melles, Kulbe, Verkulich, Pushina, Hubberten and Ricci1997). Diatom, as well as stable isotope data indicate that marine conditions prevailed in Izvilistaja Inlet (core PG1180) from prior to 7.9 ± 0.3 ka bp until 1.4 ka bp and since 9.6 ± 0.2 ka bp in Paz Cove (cores PG1173, PG1170) (Fig. 8a–c). This shows that Cacapon Inlet as well as Transkriptsii Gulf were connected to the open ocean and probably to each other, as the parallel δ13C trends in the marine cores indicate (Fig. 9). An advance and thickening of the Edisto Glacier ice tongue may have reduced water exchange with the ocean underneath the floating parts of the glacier when the grounding line advanced towards the coring locations. This probably occurred around 7.7 ± 0.2 ka bp, when brackish/benthic C. pinnata were high in PG1180 (Fig. 9). A decrease in Chaetoceros rs. in Paz Cove (core PG1173) between 7.9 ± 0.1 ka bp and 5.7 ± 0.3 ka bp could also reflect freshening due to basal melt and melting icebergs from increased calving into the bay (Fig. 9). After 7 ± 0.1 ka bp, the open ocean species F. kerguelensis is almost absent in core PG1173. This shows that connection to the ocean underneath the ice shelf or Denman Glacier became more restricted than during the early Holocene. The advance was probably not very extensive, since marine conditions continued in Transkriptsii Gulf and Cacapon Inlet (Fig. 8b). A second, late Holocene advance of Apfel Glacier and a possible expansion of the Edisto Glacier tongue into Transkriptsii Gulf and Cacapon Inlet probably occurred at 2.2 ± 0.1 ka bp, when circulation of marine waters into Izvilistaja Inlet started to decline and a sudden decline in phytoplankton productivity and a decrease in Chaetoceros blooms occurred in Paz Cove (Fig. 9). The shift in the biogenic records of the marine inlets is not well represented in the lacustrine records, pointing to changes that mainly affected the marine environment. In order to correlate our lake and marine core records with the moraine ridges described by Augustinus (Reference Augustinus2002), better age constraint of the geomorphological features is necessary. The late Holocene glacier advance we suggest was probably more extensive than the advance in the early to mid-Holocene, since marine connection of Transkriptsii Gulf became more restricted. However, freshening of Transkriptsii Gulf was probably also supported by lowering of RSL (Verkulich et al. Reference Verkulich, Pushina, Sokratova, Melles, Hultsch and Diekmann2007).
Relative Sea Level changes
Evidence for RSL change during the early Holocene comes from PG1180 in inner Izvilistaja Inlet, which is connected to Transkriptsii Gulf across a sill, which is 3 m below current sea level. RSL must have been higher than 3 m below present day sea level since at least 7.9 ± 0.3 ka bp to allow marine water to enter Izvilistaja Inlet. This site could provide important information on an early Holocene sea-level rise similar to coastal oases in Prydz Bay (Hodgson et al. Reference Hodgson, Whitehouse, DeCort, Berg, Verleyen, Tavernier, Roberts, Vyverman, Sabbe and O'Brien2016). However, due to a lack of age information of the proglacial sediment unit in this core no reliable RSL information can be derived from this earlier part of the record.
A RSL curve for Bunger Hills has been established based on raised beaches and incorporated marine fossils and diatom records (Colhoun & Adamson Reference Colhoun and Adamson1992, Verkulich et al. Reference Verkulich, Pushina, Sokratova, Melles, Hultsch and Diekmann2007, Poleschuk & Verkulich Reference Poleschuk and Verkulich2014). Saline water in Jaw Lake indicates a RSL maximum at or above 10 m a.s.l., however, the poorly dated sediment record from Jaw Lake does not allow determination of the timing of this level (Roberts et al. Reference Roberts, McMinn and Zwartz2000). A marine ingression in Algae Lake was discussed (outlet sill at c. 10 m a.s.l.), since fragments of marine diatom frustules were found in cores 6069, 6078 and 6082 (Melles et al. Reference Melles, Kulbe, Verkulich, Pushina, Hubberten and Ricci1997, Verkulich et al. Reference Verkulich, Melles, Hubberten and Pushina2002). Geochemical data (high δ13C values, no halite) throughout the records as well as the dominance of freshwater diatom species in the biogenic sediments (Verkulich et al. Reference Verkulich, Melles, Hubberten and Pushina2002), however, point to deposition under continuously fresh conditions. Water flowing from Transkriptsii Gulf into Algae Lake via Izvilistaja Inlet may not have led to a pronounced marine signal in the sediments of Algae Lake, when filled with freshwater as at present day. However, the diatom record from Izvilistaja Inlet shows marine conditions until 2.2 ± 0.2 ka bp, which postdates a proposed mid-Holocene sea level high stand. Marine diatom frustules in the Algae Lake record are not restricted to a specific period during the Holocene and could be from aeolian supply from Cacapon Inlet, since strong winds transported sea spray across the adjacent land areas (Gore & Leishman Reference Gore and Leishman2020).
The diatom record from Paz Cove (PG1173) indicates a decrease in open ocean species (F. kerguelensis, Fig. 6) after c. 6 ka bp, which could indicate a successive decrease in water exchange underneath Denman Glacier and Shackleton Ice Shelf and may be an effect of lowering RSL (Verkulich et al. Reference Verkulich, Pushina, Sokratova, Melles, Hultsch and Diekmann2007, Poleschuk & Verkulich Reference Poleschuk and Verkulich2014). Lower connectivity after 6 ka bp is consistent with a RSL high stand at around 5.5 to 6.4 ka bp with subsequent sea level fall afterwards as reconstructed from raised beaches (Colhoun & Adamson Reference Colhoun and Adamson1992). RSL lowering probably led to increasing freshwater influence in Izvilistaja Inlet (PG1180) after 2.2 ± 0.2 ka bp resulting in permanently fresh conditions from 1.4 ± 0.1 ka bp (Fig. 9). However, since the hydrology of the epishelf lakes is also affected by the dynamics of the surrounding glaciers, it is difficult to extract a clearly sea level driven signal.
Evidence for climatic conditions
In the early Holocene, climate conditions were probably relatively warm, which promoted the deglaciation of Bunger Hills and is consistent with other reconstructions from East Antarctic coastal areas, marine records and ice cores (Denis et al. Reference Denis, Crosta, Barbara, Massé, Renssen, Ther and Giraudeau2010, Verleyen et al. Reference Verleyen, Hodgson, Sabbe, Cremer, Emslie and Gibson2011). An increase in sea ice diatoms from 9.1–8.5 ka bp in core PG1173 indicates a short term cooling, but could also be explained by more persistent sea ice in freshened surface waters under high freshwater run-off (Fig. 9).
The widespread onset of biogenic sedimentation in lakes in southern Bunger Hills around 7.9 ± 0.1 ka bp indicates a decrease in meltwater run-off and associated decrease in high input of siliciclastic matter into the lakes, which allowed lake bottoms to be colonized by benthic organisms. The decrease in meltwater run-off could indicate colder conditions and reduced surface melt, but could also reflect the advanced state of deglaciation in southern Bunger Hills. From 7.9 ± 0.1 to 5.7 ± 0.2 ka bp, benthic communities in Algae Lake and 8 m lake were dominated by mosses, which are the first to colonize formerly proglacial environments, however, they also indicate low mineralisation of the water column and oligotrophic conditions (Priddle & Heywood Reference Priddle and Heywood1980). Enrichment in S in the Algae Lake sediments could indicate restricted water-column mixing and more persistent lake-ice cover related to cooler climate during that time. However, other indications for cooler climate conditions are equivocal. 17 m lake on Geographers Island filled with water around 6.9 ± 0.4 ka bp, which could be due to the initial deglaciation of the site, or enhanced snowfall and the formation of snow fields providing meltwater to the lake basin, which is inconsistent with cold climate. In the marine record (PG1173) sea ice diatoms are of low abundance from 8.5 to 6 ka bp and show only a short increase from 6 to 5.5 ka bp, which argues against a period of pronounced colder conditions (Fig. 9).
After 5.7 ± 0.2 ka bp, δ13C records from Algae Lake and 8 m lake indicate relatively stable conditions and probably warmer temperatures (Fig. 9). While high δ13C values in Algae Lake indicate high contributions of microbial mats, probably related to thin and less persistent lake ice, low δ13C values in 8 m lake indicate dilute water salinity, probably due to permanent run-off from snow fields in the catchment that balance water loss by evaporation. At the same time, sea ice diatoms decreased (PG1173), TOC increased and higher δ13C values in Izvilistaja Inlet and Paz Cove point to increased productivity, which could reflect warmer conditions (Fig. 9). The period from 4 to 1.8 ka bp probably was the climatic optimum in Bunger Hills, indicated by high marine productivity, marine water exchange between Transkriptsii Gulf and Cacapon Inlet (reduced extent of Edisto Glacier ice tongue until 2.2 ka bp) and high nutrient input in 8 m lake, which probably resulted from a thicker active (seasonally thawed) layer in sediment in the catchment (Fig. 9).
The drop in TOC and δ13C in core PG1173 at 2.2 ka bp was previously interpreted as an abrupt cooling (Kulbe et al. Reference Kulbe, Melles, Verkulich and Pushina2001). However, this shift clearly predates a late Holocene increase in sea ice diatoms at 1.7 ± 0.1 ka bp (Fig. 9). The decrease in δ13C is recorded in most marine cores (PG1180, PG1173, PG1171) and could also reflect an advance of Edisto Glacier ice tongue (see previous paragraphs). Lakes were also affected by changing environmental conditions, which resulted in desiccation of 17 m lake after 2.1 ± 0.4 ka bp and lower δ13C values in Algae Lake and 8 m lake around 1.5 ka bp, which points to lower productivity and/or lower contribution of microbial mats, probably due to thicker lake ice. The increase in sea ice diatoms and thicker lake ice indicate a cooling in Bunger Hills. A cooler and drier climate was probably followed by warming around 0.5 ka bp as indicated by refilling of 17 m lake and recurring microbial mats in 8 m lake (Fig. 9). A shift from drier to wetter (colder to warmer) conditions is also reflected in a diatom-based salinity record from Jaw Lake (Fig. 1), which shows a decrease in salinity in the late Holocene (Roberts et al. Reference Roberts, McMinn and Zwartz2000). Conditions were probably variable during the last centuries since δ13C values show large variability in the lakes (Fig. 9).
The relatively short period of cooler conditions we suggest for Bunger Hills is in contrast to reconstructions from marine records from Prydz Bay and Wilkes Land, which show a cooling with more sea ice since 4 ka bp (Denis et al. Reference Denis, Crosta, Barbara, Massé, Renssen, Ther and Giraudeau2010). Other coastal oases in East Antarctica also experienced cooling during the past two millennia and some were also affected by relatively short-term drying/cooling events (Verleyen et al. Reference Verleyen, Hodgson, Sabbe, Cremer, Emslie and Gibson2011). A cooler climate on land was probably a result of atmospheric cooling over the continent as recorded in ice cores (Crosta et al. Reference Crosta, Crespin, Swingedouw, Marti, Masson-Delmotte, Etourneau, Goosse, Braconnot, Yam, Brailovski and Shemesh2018). A late Holocene grounding line advance of Apfel and Edisto glaciers could be associated with cooling on land. However, Denman/Scott Glacier and Shackleton Ice Shelf also respond to changes in basal melt rates, which have been increasing since c. 4 ka bp around Antarctica and were probably promoted by intrusion of warmer water subsurface waters on the shelf (Crosta et al. Reference Crosta, Crespin, Swingedouw, Marti, Masson-Delmotte, Etourneau, Goosse, Braconnot, Yam, Brailovski and Shemesh2018). The advance of Apfel and Edisto glaciers probably occurred in response to basal melting and thinning of the ice tongue prior to 2.2 ka bp.
Conclusions
Age-depth models for existing records from epishelf and inland lakes in Bunger Hills reveal common regional patterns of environmental changes for the Holocene epoch. While the timing of deglaciation was well known from the onset of biogenic sedimentation in inland lakes and epishelf lakes in the early Holocene (around 9.6 ka bp), the re-evaluation of records from Algae Lake, 8 m lake and Izvilistaja Inlet now show that intensive melting and discharge of clastic matter from the ice sheet continued until c. 7.9 ka bp. After that, only local ice melt, probably from remnant ice or snowfields, affected the inland lakes, which is in agreement with previous findings of a relatively stable ice sheet margin after the early Holocene. In contrast, the epishelf lakes in Bunger Hills responded to changes in grounding line position linked to glacier mass balance and/or RSL change. The records show that the inner parts of Bunger Hills were connected to marine waters since at least 9.6 ka bp, pointing to grounding line positions of Denman/Scott glaciers and Shackleton Ice Shelf that allowed marine water to reach the present-day coast. Based on diatom assemblages and geochemical data we infer at least two periods of advances of Edisto and Apfel glaciers for the Holocene. The first advance probably occurred around 7.7 ± 0.2 ka bp after a period of relatively free water exchange between the epishelf lakes and the ocean. Around 2.2 ka bp a drop in marine productivity and successive freshening of Izvilistaja Inlet afterwards could have resulted from grounding line advance into Transkriptsii Gulf. However, to distinguish effects of RSL from climate/ocean driven glacier dynamics, further studies are necessary to better i) constrain the RSL history of Bunger Hills (e.g. with new records from isolation basins), ii) quantify possible thinning and mass loss of the glaciers (e.g. from terrestrial records from Bunger Hills and other outcrops from the Denman/Scott glacier system) and to, iii) confirm the timing and intensity of meltwater discharge into the Southern Ocean (e.g. from marine records from the East Antarctic margin).
Although the records considered here display common trends, it is difficult to extract a clear climate signal based on the proxies considered. Some biogenic signals, which were previously interpreted in terms of climatic conditions, could also be explained by a natural evolution of the lakes after ice retreat (such as successive enrichment in nutrients from progressive rock weathering in the catchment and input of sea spray, or stabilisation of benthic habitats). Other, short-term (millennial to centennial) fluctuations are difficult to correlate between records, due to uncertainties in the age models. Nevertheless, the synchronicity of changes in the biogenic records of lakes and marine inlets point to regional relevance of environmental changes, which could indicate climatic forcing.
Acknowledgements
M. Melles received funding by the DFG, Deutsche Forschungsgemeinschaft (Grant no. ME 1169/1). Data published in Kulbe (Reference Kulbe1997) is freely accessible at www.pangaea.de. We thank two anonymous reviewers and Prof. Mike Bentley for constructive comments on a previous version of the manuscript.
Author contributions
SB conceived the paper and led the writing of the manuscript. All authors contributed to the discussion and interpretation and in drafting and editing the article.