NOMENCLATURE
- CO
Carbon monoxide
- CO2
Carbon dioxide
- FAA
Federal Aviation dministration
- FDS
Fire Dynamics Simulator
- HRR
Heat release rate (kW)
- LT
Light transmission (%/m or %/ft)
- NIST
National Institute of Standards and Technology
- PHRR
Peak heat release rate (kW)
- ULD
Unit load device
- I
Photocell raw voltage
- I 0
Initial photocell raw voltage
- L
Total path length
- O
Smoke Obstruction (%/m or %/ft)
1.0 INTRODUCTION
Fire safety is of paramount importance for aircraft, and particularly for advanced and future transport aircraft where metals are replaced with lightweight but more combustible polymer composite materials (A350, B787)(Reference Branch1). One critical area is the cargo compartment, which is mostly inaccessible during flight but can contain combustible materials such as Lithium-ion batteries inside luggage. Although in-flight fires are rare, the consequences of a fire can be very problematic.
FAA, along with regulatory agencies throughout the world, require cargo compartments be equipped with effective fire detection and suppression systems in order to warn, suppress and control fire incidents(2,3) . At present, the fire detection and suppression systems are installed with fire detectors being placed at the ceiling of the cargo compartment and fire extinguishing bottles being carried on board to deluge flood the whole cargo compartment with suppression agents in case of a fire incident(Reference Hipsher and Ferguson4).
Although a cargo compartment-based fire detection and suppression system has the advantage of one system in a fixed position and hence is easy to check and maintain, there are a number of disadvantages. Fires on aircraft usually start from luggage inside cargo containers, also named unit load devices (ULDs), which are used to load and move passenger baggage, air cargo and mail. Thermal heat runway from a failure battery in a passenger luggage could potentially initiate a fire and further ignite the surrounding combustible materials such as fabric, polymer and cardboard(Reference Branch1,Reference Wang, Ping, Zhao, Chu, Sun and Chen5-Reference Ribiere, Grugeon, Morcrette, Boyanov, Laruelle and Marlair8) . Because the fire is inside the container, very little smoke is leaked out to the outside cargo compartment. For the cargo compartment-based fire detection system to work, the fire detection threshold is set at a very sensitive level(Reference Lu, Mao, Wang and Lu9,Reference Girdhari10) to ensure safety. Otherwise, by the time the cargo detector has sensed a fire, the fire inside the container may have already grown to a dangerous level, e.g. flashover, which would make fire suppression difficult and sometimes impossible. However, setting a very sensitive detection threshold causes large numbers of false alarm because the smoke detector system can be easily triggered by nuisance sources such as small airborne particles. According to(Reference Blake11), the ratio of false alarm to detecting actual fire in cargo compartment-based smoke detection system is about a hundred to one. Because the cargo compartment is non-accessible, false alarm can impose a severe toll, the consequence of which may include flight diversion, declaration of emergency situation that eventually leading to passenger evacuation, compartment inspection, fire extinguisher replacement, customer and passenger disappointment, and loss of confidence in the warning system(Reference Girdhari10). Furthermore, a cargo-based fire suppression would require carrying a large amount of fire suppression agent and would cause a lot of damage when released to flood the entire cargo area.
A container fire detection and suppression system would overcome the above shortcomings of the cargo-based system, allowing the fire to be rapidly detected closest to its source, and controlled and suppressed inside the container. Most importantly, due to strong fire signals near the fire source, a container-based fire detection system allows a high fire-detection threshold to be set, thereby completely eliminating or drastically reducing false alarm. With advances in electronics and wireless communication, a container-based fire detection system is eminently feasible.
This paper presents the analysis of a container-based fire detection and suppression system. It first thoroughly explains aircraft fire safety requirements and current fire protection technologies to identify different possible aircraft fire scenarios and qualitatively assess possible solutions. The computational fluid dynamic model for fire safety (FDS, standing for Fire Dynamics Simulator)(Reference McGrattan, Hostikka, McDermott, Floyd, Weinschenk and Overholt12) is then used to predict fire development inside a typical container under a range of fire scenarios. The simulation results are used to identify the worst fire scenario and to select the optimum placement of fire detector sensors, to set the threshold for sensor activation, and to inform container requirement such as airtightness for effective fire suppression.
2.0 FIRE PROTEXTION FOR CARGO COMPARTMENT
According to the certification specification rule CS25.858(2), a cargo compartment is required to be equipped with a detection system to provide active fire protection. The system provides an aural and visual indication to the flight crew in the early stage of fire prior to it breaking out into a large fire. The system must be capable of detecting a fire at a temperature significantly below that at which the structural integrity of the aeroplane is substantially decreased. Specifically, the fire must be detected within 1 minute of fire occurrence in the cargo. The other operational and certification requirements include means to allow the crew to check the functioning of each smoke or fire detector circuit in flight. Effectiveness of the detection system must be shown for all approved operating configurations and conditions.
For fire suppression, the current practise of cargo-based active fire suppression system defines a minimum performance standard(Reference Reinhardt13), which requires a minimum agent concentration level to adequately protect an aircraft cargo compartment against fire. Other general requirements are that any fire inside the cargo must be contained, the temperature rise must not adversely affect integrity of the structures and smoke generated inside the cargo compartment cannot cause hazard to the occupied areas. For other suppression options such as a container suppression system, the development of a specific certification and standard are still ongoing.
2.1 Current fire detection system for cargo compartment
Smoke, heat, CO and CO2 are the major quantifiable fire signatures, and fire detectors use these signals to detect early fires. Even though fire signatures from various cargo goods differ significantly in terms of smoke, gas production and heat release, for a cargo compartment detection system, smoke detectors are often preferred because smoke detection can cover a wide range of scenarios from smouldering fire to flaming combustion(Reference Girdhari10,Reference Grosshandler14) .
Smoke detectors sense the aerosol produced from combustion. Almost all cargo compartment smoke detectors are based on photoelectric sensing. In this system, smoke particles interfere with a light beam inside the detector, causing the light to scatter onto a photosensitive diode, which increases the photodiode’s current output and generates an alarm once a threshold is exceeded. The threshold for photoelectric sensors is set in terms of reduction in light transmission. Light transmission (LT) is calculated as follows(Reference McGrattan, Hostikka, McDermott, Floyd, Weinschenk and Overholt12):

where L is the total path length and I and I 0 are photocell raw voltage and the initial photocell raw voltage.
This method of fire detection using smoke light transmission has been the technology of choice by the aircraft manufacturing industry for the last three decades(Reference Blake and Suo-Anttila15). In addition, the FAA requires that detectors meet standards referenced in SAE Aerospace Standard (AS) 8036(Reference Bell16), which specifies that the alarm must be raised when light transmission decreases to 96%/ft, i.e. the reduction in light transmission is 4%/ft. However, this threshold setting is highly sensitive and can be easily triggered by the presence of mist and dust thereby causing false alarm. For example, according to(Reference Girdhari10), dust can cause light transmission to decrease by 9%/ft to 91%/ft. Previous studies have focused on reducing false alarm rate by combining multiple fire signals(Reference Chen, Hovde, Peterson and Marshall17), however, setting a complex criterion for the detection system risks delaying alarm or not detecting fire.
2.2 Cargo-based fire suppression system
The current cargo-based suppression system applies the total flooding method using the gaseous agent Halon 1301 (Bromotrifluoromethane CBrF3), although the production of this chemical was banned in 1994(Reference Blake and Atlantic City18). Many suppression bottles are installed in the cargo compartment. The first step in controlling and suppressing a fire is shutting down airflow to the cargo hold. Then the fire suppression system provides Halon 1301 with a minimum concentration of 5% to cover the entire cargo compartment for a specified duration depending on the airplane model, to suppress the fire for a sufficient time until the airplane lands at the nearest airport.
This suppression system cannot deal with a specific container under fire attack and therefore requires a significant amount of extinguishing agent to be stored for the whole cargo hold. The suppression bottles, distribution pipes and fire resistant liners add a substantial amount of weight to the aircraft even when the cargo is not loaded with luggage containers. Moreover, it has a high maintenance cost. A container-based suppression system would overcome these shortcomings. Furthermore, it may be possible to employ passive fire suppression by starving oxygen supply to the container fire, although this only works if the amount of air supply to the container through leakage is kept to relatively low level.
In summary, there is a need to develop a container-based fire detection and suppression system that can significantly reduce false alarms, improve alarm time response and has in situ fire suppression ability. This requires careful consideration of different fire scenarios, feasibility, effectiveness and installation and communication of fire detection sensors, feasibility of a container-based suppression system.
3.0 NUMERICAL MODELLING OF CONTAINER-BASED FIRE DETECTION SYSTEM
In this section, the Fire Dynamics Simulator (FDS)(Reference McGrattan, Hostikka, McDermott, Floyd, Weinschenk and Overholt12) is used to analyse fire development and smoke transportation inside a container and to record activation response of fire detectors to different fire signatures so as to identify the shortest detection time. The study uses the LD3-45 container designed for the A320-family. This container has an internal space of 3.7m3(19) with the dimensions shown in Fig. 1. Based on consideration of limited internal volume, it is suggested that a single detector will be installed per container. In order for the single detector to be successful, it should be able to detect fire in less than 60 seconds for any possible fire scenario.
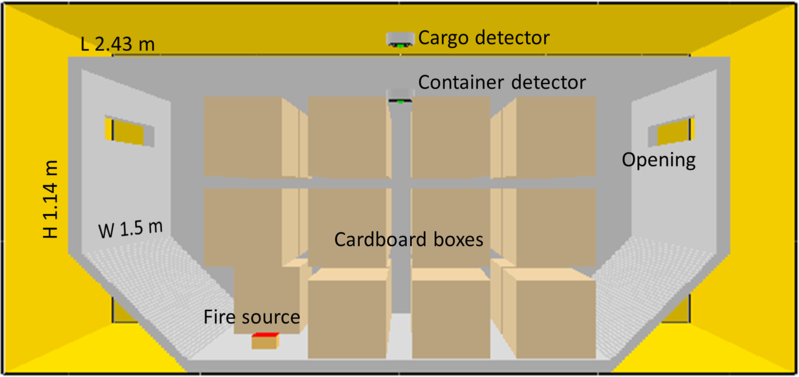
Figure 1. Fire simulation model for an air cargo container.
3.1 Fire dynamics simulation model
The Fire Dynamics Simulator (FDS) is a computational fluid dynamics (CFD) model for fire-driven fluid flow, developed by the National Institute of Standards and Technology (NIST) of the United States Department of Commerce. It numerically solves a large eddy form of the Navier-Stokes equation. It is specifically developed for low-speed, thermally driven flow, with emphasis on smoke and heat transport during fire development. FDS is open source and is continually updated by international researchers to incorporate the latest advance in understanding of fire dynamics. It is also widely employed by the fire protection industry. Its validity for simulation of smoke, heat, and gas species transportation has been confirmed by numerous studies(Reference Lu, Mao, Wang and Lu9,Reference Blake and Suo-Anttila15,Reference Oztekin20-Reference Chen, Shao and Yang22) .
FDS does not directly calculate smoke obstruction (light transmission). Instead, smoke obstruction is calculated by integrating soot concentration in the light beam path using Beer’s Law(Reference Blake and Suo-Anttila15):

where σs is the specific extinction coefficient (7400m2/kg), Csoot is soot concentration (kg/kg), and ρcell is gas density (kg/m3).
Although FDS is mainly used for assessment of fire safety in buildings, it has also been used in aerospace applications. For example, Blake et al.(Reference Blake and Suo-Anttila15) employed FDS to simulate behaviour of a forward cargo compartment fire inside a Boeing 707, with particular interest in ceiling temperature, light transmission and gas concentration. They obtained FDS simulation results in close agreement with experimental results provided by FAA(Reference Suo-Anttila, Gill, Luketa-Hanlin and Gallegos23). Their case study forms part of the validation of FDS and their input data (smoke generation rate, CO and CO2 productions rates) are listed in the FDS manual(Reference Suo-Anttila, Gill, Luketa-Hanlin and Gallegos23). The analysis presented in this article uses the same input data(Reference McGrattan, Baum, Rehm, Hamins and Forney24). The authors made this decision because many different types of materials may be found inside a container in realistic situations and the generic fire source developed for the FAA project is considered the most appropriate to replicate a cargo fire.
Figure 1 shows a typical FDS computational domain for the cargo space with a container, consisting of approximately 212,400 cells with cell sizes in the range of 0.01 to 0.05m. When simulating smoke transportation in an enclosed container, various conditions should be considered to replicate various possible fire scenarios. To identify the worst case scenario for fire detection, the following factors are considered.
3.1.1 Fire source
Typical combustible materials inside an aircraft cargo container can be of a great range, including battery, leather, fabrics, cardboard and household plastics. Among them, lithium-ion battery presents the highest fire risk and numerous fire accidents in aircraft are caused by overheating or spontaneous combustion of such batteries(Reference Wang, Ping, Zhao, Chu, Sun and Chen5). This is considered as the fire source in this research.
To find a representative fire source and to eliminate any ambiguity about the start time of the fire, the FAA technical centre has developed a standardised fire source(Reference Blake25), consisting of plastic resin pellets to produce a consistent, representative, and repeatable fire. This fire source produces a similar quantity of smoke as in aircraft smoke detection certification tests. Moreover, the heat and other combustion gases produced from this fire source are representative of actual fires. Therefore, it has been proposed as the standard to be used for cargo fire detection in the aviation industry(Reference Blake and Suo-Anttila15,Reference Blake25) .
The same fire source is adopted in this research, represented by a 0.1m × 0.1m burner tray (Fig. 1) inside the container producing a peak heat release rate (PHRR) of 5 kW as shown in Fig. 2. The heat release rate from ignition to 100s is used in the FDS model to replicate the start of a typical fire incident.

Figure 2. Heat release rate (HRR) from lower-bound, standardised and upper-bound fire sources(Reference Ribiere, Grugeon, Morcrette, Boyanov, Laruelle and Marlair8,Reference Blake25) .

Figure 3. Fire locations at bottom layer of the container.
However, the actual rate of heat release of a lithium battery fire may be higher or lower than the standardised fire source recommended by FAA in Fig. 2. Therefore, to test fire detection effectiveness for a range of battery fires inside the container, a lower bound (2.6 kW) and an upper bound (13kw) PHRR are obtained, based on cone calorimeter testing results of various batteries(Reference Ribiere, Grugeon, Morcrette, Boyanov, Laruelle and Marlair8). In addition, battery fire test results indicate that they have higher rates of HRR values than proportionally scaling the standardised fire source using ratios of PHRR, as is done for the lower and higher bound curves in Fig. 2. Therefore, the lower and upper bound curves in Fig. 2 give conservative estimates (lower values) of heat release rate before reaching PHRR values. This is intentional because a lower value of HRR makes the fire detection time longer, thus is a worst case scenario for fire detection that will generate the lowest fire signal, thus requiring the longest time to detect.
3.1.2 Fire source location
A fire can occur anywhere inside the container. In this research, three locations at the bottom of the container (Fig. 3) in each quarter are considered: at the centre (Burner 1), near the corner (Burner 2) or along the edge (Burner 3). Since the fire detector will be placed at the ceiling level, assuming the fire source to be at the bottom of the container is the worst case scenario as it would take the longest time for fire signal to travel to the fire detector and hence detect.
3.1.3 Container loading
The loading inside the container affects smoke transportation behaviour: a sparsely loaded container has more empty space and more air supply for smoke production, but may dilute smoke signals. On the other hand, a densely packed container has low air supply, but may delay smoke transportation. Therefore, two extreme situations—an empty container and a fully loaded container with cardboard boxes (Fig. 1)—are considered.
3.1.4 Leakage of container
In general, a container is not completely sealed and has some air leakage. Since the volume inside a container is limited, air leakage is an important source of air supply. The exact amount of air leakage in the Airbus LD3-45 container is not known, but a value of 0.1m2 is considered as a reasonable estimate for this parametric study. This value is equivalent to a slit of 0.014m wide along one side of around 7m of the container edge. The oxygen supply and pressure is set at the sea level and ambient environment.
3.1.5 Location of the fire detector
The time taken for a fire to be detected depends on the relative spatial arrangement of the fire source and the fire detector. Therefore, for each fire source location, fire detectors at various locations are assessed. In FDS simulation, the virtual fire detector can be placed anywhere—they are merely locations where fire signals are output. A total of 25 detectors at the container ceiling level are considered and they are shown in Fig. 4. Gas temperature, smoke light transmission, CO and CO2 concentrations are continuously recorded for each simulation. Due to symmetry of the rectangular container, the detectors can be categorised into nine different location groups as marked by different colours in Fig. 4. One extra detector (see Fig. 1) is placed outside the container on the ceiling of the cargo compartment for assessment of effectiveness of cargo-based detection.
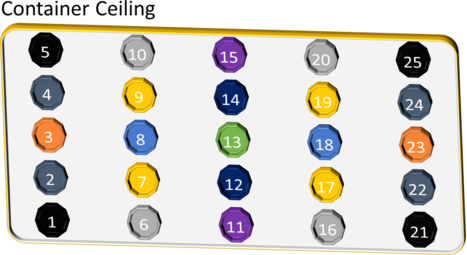
Figure 4. Fire detector candidate locations at the container ceiling level.
Thus, there are 12 simulation cases, consisting of 3 levels of rate of heat release (lower bound, standardised, upper bound), 2 container loading conditions and 2 container leakage rates (0 and 0.1m2).
3.2 Analysis of simulation results
The fire inside a container could start at any location, so the best compromise is to install the single detector at the centre of the container ceiling. Nevertheless, it is useful to evaluate the performance of fire detectors at different locations so that an area of the ceiling may be considered to be suitable allowing flexibility of installation of the fire detector. Figure 5 shows the range of fire signals reached at the different detectors at 60 seconds, for the standardised fire source. Detector 13 is at the ceiling centre.

Figure 5. Range of fire signals from different sensor locations at 60s after fire ignition.
Use temperature signal as example. As expected, temperatures at other detectors can be higher than that at the ceiling centre, depending on the fire location. However, when setting the threshold of a detector, the threshold should be as high as possible so as to reduce alarm by environmental nuisance, but should be as low as possible so as to detector fire from any fire location. This means that the weakest signal (minimum value) detected by the detector should be as high as possible. Figure 5 shows that as expected, the ceiling centre detector (detector 13) has the best performance: Its weakest fire signal (40.9 oC) is higher than at all other detector locations. Nevertheless, the fire detectors within the central quarter of the container ceiling (detector groups 12 & 14, 8 & 18, 7, 9, 17 & 19) also have high minimum fire signals, indicating that the single fire detector can be located within the central quarter if practicality prevents the single detector from being placed at exactly the ceiling centre. Other fire signals (smoke, CO and CO2) share the same conclusion as the central sensor outperformed other locations considering all case scenarios.
To identify the best signal for fire detection, being defined as the signal that takes the shortest time to be detected, Fig. 6 displays the four fire signatures received from the central ceiling detector in various scenarios under the standardised fire. The results show that smoke obstruction (or reduction in light transmission, %/m) takes the shortest time to reach a much higher level than environmental exposure. For comparison, a temperature of 40°C may be considered an easily reachable environmental condition so the setting of a temperature detector would need to be higher than this value. Yet, it would take a long time for a temperature of 40°C to be detected. Also, CO/CO2 levels depend on the input assumption of yield of the combustible materials, whilst the input data for smoke obstruction is generally not very sensitive to different combustible materials(Reference Grosshandler14,Reference Behle26) . Furthermore, Fig. 6 also shows that the smoke obstruction values are within a narrower band than other fire signatures. This indicates that using a smoke obstruction detector would have the least variability in detecting fire and would thus have the most consistent performance.

Figure 6. Range of fire signals at central ceiling detector. (a) Smoke obstruction; (b) Temperature; (c) CO concentration; (d) CO2 concentration.
If it is necessary to conduct a physical fire test for certification of the fire detection system, it is also required to identify the worst case for fire detection, i.e. the case which would take the longest time to detect the fire. Figure 7 shows the effects of different simulation parameters on smoke obstruction value for the standardised fire.

Figure 7. Smoke signals at central ceiling detector under different scenario parameters: (a) Fire location; (b) Container loading; (c) Container leakage.
Figure 7(a) clearly shows that the corner fire takes the longest time to reach the smoke detector at the ceiling centre. When the container is fully loaded (Fig. 7(b)), the smoke signal is intially (before 30s after ignition) lower than in the case of an empty container, because the smoke transportation is blocked by piled cardboard boxes, and therefore has a more tortuous route to diffuse inside the container. However, afterwards, the fully loaded container has slightly higher smoke concentration than the empty container because the amount of air inside the fully loaded container is limited. If the container has leakage (Fig. 7(c)), the smoke obstruction value is almost identical to that in the sealed container during the very early stage of the fire because there is sufficient air supply inside the container and smoke transportation happens inside the container. Afterwards, the smoke obstruction level inside the container with leakage becomes lower than that inside the sealed container because of leakage of smoke to the outside.
Therefore, for certification purposes, it is suggested to conduct a test in an empty, unsealed container, with a corner fire source and heat release rate at the lower bound of a lithium battery fire.
4.0 ASSESSMENT OF CONTAINER-BASED SMOKE DETECTOR
Having chosen to install a central ceiling smoke obstruction detector, it is necessary to determine the maximum level of smoke obstruction that can be set to ensure smoke detection within 60 seconds for all possible fire sizes. For this purpose, in addition to the standardised fire source, the other two fire sizes in Fig. 2 (lower bound and upper bound HRR curves of lithium batteries) are considered.
4.1 Threshold of container-based smoke detector
Figure 8 compares smoke detector performance inside and outside the container, with smoke obstruction being set at 1, 2, and 3 times of the standard nominal cargo detector alarm level of 4%/ft or 12.5%/m(Reference Girdhari10). The conversion of smoke obscuration from units of %/ft to %/m is given by(Reference McGrattan, Hostikka, McDermott, Floyd, Weinschenk and Overholt12):

Note here it is assumed that the leakage opening is close to the container roof as shown in Fig. 1 and only a partial cargo space around the container is simulated; therefore, the outside smoke detector registers the strongest fire signature. Should the container leakage be elsewhere, for example on one of the vertical sides such as the door way, which is most likely, or should the whole cargo space be simulated, which will have more space to further dilute the leaked smoke, the outside smoke detector would be less efficient.
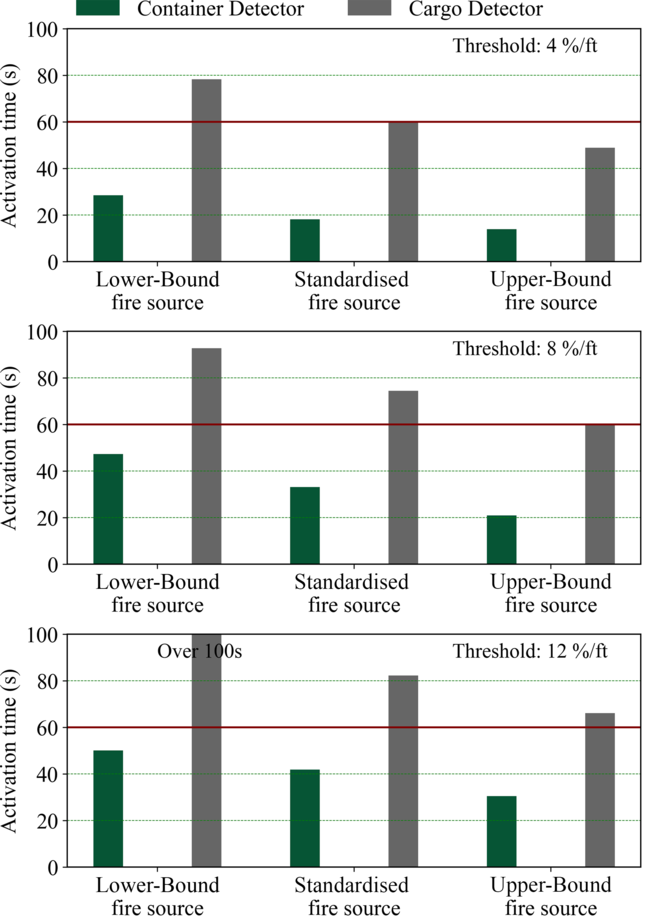
Figure 8. Comparison of activation times of cargo and container detectors for different smoke obstruction thresholds under three different fire sources.
The results in Fig. 8 indicate that the cargo detector is just about to detect the standardised fire at 60 seconds if the smoke obstruction threshold is set at the nominal value of 4%/ft. However, it would miss the fire detection target with a lower rate of heat release. In contrast, the container detector can detect even the lower bound fire before 30 seconds. If the smoke obstruction threshold is set at twice the nominal value, at 8%/ft, the cargo detector can only detect the upper-bound fire within 60 seconds, whilst the container detector can detect the lower-bound fire within 50 seconds. Even when the smoke obstruction threshold is set at 3 times the nominal alarm level, the container detector is still able to detect the fire well before 60s, whilst the cargo detector would miss the fire detection target for all fires.
4.2 Assessment of false alarm
Reducing false alarms is one of the main objectives of developing a container-based fire detection system. To quantify the effectiveness of this detection system, the nuisance sources such as mist and dust, which are the main causes for false alarms, need to be analysed.
The Arizona dust test was designed to simulate particulates that could be present within cargo compartments and one test reported a peak smoke obstruction value of 9%/ft(Reference Oztekin, Blake and Lyon21). This is well above the standard alarm level of 4%/ft, which explains the present high ratio of false alarms (>90%). If the threshold for container detector is set at 12%/ft, as explained in the previous section, it would appear that false alarm could be totally eliminated.
However, it is possible that the environmental particulate level may be higher than the reported peak value of 9%/ft with a small probability of occurrence. Due to a lack of detailed data of the level of environmental exposure such as mist and dust, precise quantification is not feasible. Nevertheless, it is possible to estimate the likelihood of being successful in eliminating false alarm based on statistical analysis.
The probability of environmental particulate level exceeding 4%/ft is conservatively estimated to be 90%, based on the reported false alarm rate of over 90% when the alarm level is set at 4%/ft(Reference Girdhari10). The probability of environmental particulate level being higher than 9%/ft is about 10% as reported in the Arizona test(Reference Oztekin, Blake and Lyon21). These two assumptions can be expressed as follows:

Assume the distribution of environmental particulate level has a normal probability distribution.

In order to satisfy the probability conditions in Eq. (4), the mean value (
$\mu )$
and standard deviation (
$\sigma $
) can be determined to be 6.5 and 1.95, respectively. Figure 9 plots the assumed probability distribution curve for environmental particulate obstruction.

Figure 9. Assumed probability distribution of light obstruction due to environmental particulates.
Based on the assumed probability distribution in Fig. 9, if the light obstruction level is set at 8%/ft, the probability of this level being exceeded, and hence causing false alarm, is 22%. This is a significantly improved situation compared with a false alarm rate of over 90%. If the light obstruction threshold is set at 12%/ft, as proposed in the previous section, then 99.8% of light obstruction caused by environmental particulate exposure would not cause false alarm, indicating a false alarm probability of 0.2%. Compared to the present rate of false alarm of over 90%, this is a 3 orders of magnitude of improvement.
In summary, a container-based sensor, installed at the ceiling centre, with a light obstruction threshold of 12%/ft, can detect all fires within 60 seconds, while the probability of false alarm is reduced to 0.2%.
5.0 PASSIVE FIRE SUPPRESSION SYSTEM FOR A SMART CONTAINER
It is possible to extinguish fire within a container if the fire is starved of oxygen supply. This is termed passive fire suppression. If the container is fully sealed, fire extinguishment is automatic. However, it would be unrealistic for the container to be completely sealed due to high cost of construction and light in-service damage. But if the air supply is low from the leakage area, it would still be possible to extinguish the fire. This study examines the maximum leakage allowed to ensure automatic fire extinguishment. With a low leakage rate, it is possible for pressure to build up inside the container. Therefore, it is also necessary to investigate whether the overpressure could cause any problem to structural integrity of the container.
5.1 Maximum leakage size for automatic fire extinguishment
At this initial stage of developing an in-container suppression system, the specific requirements and standard for certification are not available. Therefore, design of the worst case scenario for fire suppression has to be based on an assessment of the effects of changing different design parameters, as explained below.
The worst case for passive fire suppression is when the fire is in an empty container due to the maximum supply of air. The fire location at the container floor has no effect due to small size of the container. In terms of the fire size, a small fire such as the lower-bound fire source possesses relatively low hazard before it develops to a larger fire, and the fire growing process would provide more response time for the flight crew to take measures as fire detection is able to detect the lower-bound fire almost instantly. Therefore, the key challenge is whether the proposed passive suppression system is able to control a fire that has developed to a considerable level.
Therefore, the upper-bound fire source in Fig. 2 is investigated because this is considered the worst fire scenario for fire suppression. After 100 s, it is assumed that the HRR is maintained at the PHRR until self-extinguishing is achieved. Should the actual fire be even larger than this, it will only lead to faster oxygen consumption, thus making self-extinguishing more likely. It should be pointed out that in the simulations, the container structure maintains its structural integrity. This is justified on the basis that the glass fibre reinforced composite made container is able to survive an intense fire with peak temperatures over 600°C for 4 hours(Reference Baxter, Kourousis and Wild27).
Figure 10 compares times of self-extinguishment of fire for different leakage areas. Self-extinguishment is defined as when there is no open flame(Reference Hurley, Gottuk, Hall, Harada, Kuligowski, Puchovsky, Torero, Watts and Wieczorek28). It can be seen that as expected, the container fire takes longer to die out with increasing leakage size. When the leakage size is larger than 0.01m2, the fire becomes continuous without achieving self-extinguishment. Therefore, if passive fire suppression is to be implemented, the leakage size should be no more than 0.01m2. It should be pointed out that this proposed leakage size limit would be better for container fire detection than when the leakage area is 0.1m2 as assumed in the study on fire detection. Therefore, the previous conclusions on fire detection still apply.

Figure 10. Comparison of times of self-extinguishment of fire for different leakage sizes.
As with fire detection, placing fire on the container floor would be more dangerous than above the floor. For example, Fig. 11 compares self-extinguishment times between placing the fire on the floor and at the mid-height of the container for a few leakage sizes. When the fire is at mid-height of the container, smoke concentration increases and oxygen concentration decreases, making it easier to extinguish the fire.

Figure 11. Self-extinguishing time with fire source at varying height.
5.2 Risk of overpressure
Figure 12 plots overpressure inside the container with increasing leakage size. The overpressure decreases rapidly with only a very small leakage size.

Figure 12. Overpressure inside container with varying size of leakage area.
According to the requirements for pressure equalisation during normal flight operations (ISO 11242)(29), the cargo container must have a minimum leakage of 5cm2 per cubic metre of the container internal volume. For a typical LD3-45 container with the internal volume of around 3.7m3, this equates to a minimum vent (leakage) area of 0.002m2. When the leakage area is greater than 0.002m2, the maximum overpressure is about 50Pa as shown in Fig. 12. This pressure is orders of magnitude lower than the resistance of the container, which has to resist wind pressure and very high pressure difference during emergency rapid decompression 15kPa(29). In fact, although unlikely to be necessary, overpressure in fire can be easily controlled. For example, should overpressure of passive fire protection be an issue, a pressure equalisation device such as a blowout panel can be incorporated into the container wall that can automatically spring back (shut) after the pressure has equalised and airtightness restored.
6.0 CONCLUDING REMARKS
This paper has presented the results of a detailed fluid dynamics numerical investigation to evaluate feasibility of a container-based fire detection and suppression system for aircraft cargo compartments. The study made use of a validate FDS model and the principal requirements of the fire system were to detect any container fire within 60 seconds, eliminate false alarm, and self-extinguishment of fire.
A series of numerical simulations were performed to examine the effect of fire location and ferocity, different leakage sizes, and loading conditions of the container. Based on detailed analysis of the simulation results, it was found that a container-based fire detection and suppression system is feasible and effective with one single smoke obstruction detector, placed at the centre of container ceiling. It can detect fire inside the container within 60 seconds no matter where the fire starts and for any size of the fire bigger than the lower bound of a lithium battery fire. The certification of container-based fire detection system should be based on the worst case, which is an empty container with the maximum leakage size with the lower-bound size fire in a corner of the container.
The light obstruction threshold can be set at 12%/ft, which is 3 times that of the present nominal setting of 4%/ft for cargo-based fire detection system. At this level, the rate of false alarm is 0.2%, which is 3 orders of magnitude improvement compared to the current rate of false alarm of over 90%.
Passive fire protection, by using restricting oxygen supply to the fire, is possible. If the leakage size is no more than 0.01m2, the standardised fire inside a container would self-extinguish within a few minutes. Provided the container has a minimum leakage size of 0.002m2, which is required for normal operation, overpressure inside the container is negligible compared to other pressures (e.g. wind, decompression) during normal operations of the aircraft.
ACKNOWLEDGEMENTS
This work was supported by the project of Development and Manufacturing of Intelligent Lightweight Composite Aircraft Container (Project ID: 785472), funded under: H2020-EU.3.4.5.1. IADP Large Passenger Aircraft.