Summations
-
DNA methylation (DNAm) is an epigenetic mechanism with high specificity.
-
Stress, depression, and antidepressant drugs modulate DNAm.
-
DNAm is a potential biomarker of depression and antidepressant response.
Considerations
-
Contradictory results are found in studies investigating the involvement of DNA methylation (DNAm) in stress, depression, and antidepressant responses.
-
DNAm is a specific epigenetic mechanism influenced by diverse factors making difficult the interpretation of the data.
-
The analyses differ across the studies, for example, investigating diverse tissue, region, sites, and methods.
Introduction
Environmental stressors contribute to the development and progression of several psychiatric disorders, including major depressive disorder (MDD or depression) (Kendler et al., Reference Kendler, Karkowski and Prescott1999, Hammen, Reference Hammen2005, Heim et al., Reference Heim, Newport, Mletzko, Miller and Nemeroff2008, Slavich and Irwin, Reference Slavich and Irwin2014). Epigenetic alterations have been proposed by several preclinical and clinical studies to mediate the association between these factors (Heim et al., Reference Heim, Newport, Mletzko, Miller and Nemeroff2008, Johnstone and Baylin, Reference Johnstone and Baylin2010, Sun et al., Reference Sun, Kennedy and Nestler2013, Slavich and Irwin, Reference Slavich and Irwin2014, Turecki and Meaney, Reference Turecki and Meaney2016, Pena and Nestler, Reference Pena and Nestler2018).
The term epigenetic refers to molecular mechanisms that change the gene expression patterns without any modification in the underlying DNA sequence (Allis and Jenuwein, Reference Allis and Jenuwein2016). The epigenetic modifications have been related with diverse conditions including the control of signal transduction pathways and cell lineage specification (Cortese et al., Reference Cortese, Lewin, Backdahl, Krispin, Wasserkort, Eckhardt and Beck2011, Cabrera-Licona et al., Reference Cabrera-Licona, Perez-Anorve, Flores-Fortis, Moral-Hernandez, Gonzalez-De La Rosa, Sanchez, Chavez-Saldana and Arechaga-Ocampo2021, MacArthur and Dawlaty, Reference Macarthur and Dawlaty2021). One common epigenetic mechanism is DNA methylation (DNAm). Increased DNAm, a typically repressive epigenetic mechanism that results specially in gene silencing, has been associated with stress and depression (Oh et al., Reference Oh, Chambwe, Klein, Gal, Andrews, Gleason, Shaknovich, Melnick, Campagne and Toth2013, Klengel et al., Reference Klengel, Pape, Binder and Mehta2014). Stress exposure causes specific DNAm modifications in genes associated with the neurobiology of depression, including the serotonin transporter (SLC6A4 or 5-HTT), brain-derived neurotrophic factor (BDNF), glucocorticoid receptor (NR3C1 or GR), mineralocorticoid receptor (NR3C2 or MR), FK506-binding protein 5 (FKBP5), and corticotrophin-releasing hormone receptor 1 (CRHR1) (Ding and Dai, Reference Ding and Dai2019). These modifications induce long-lasting effects on the expression of these genes, leading to brain structural and functional changes and behavioural alterations. However, it is still unclear how stress induces these DNAm changes to cause its behaviour and molecular effects (Fig. 1).
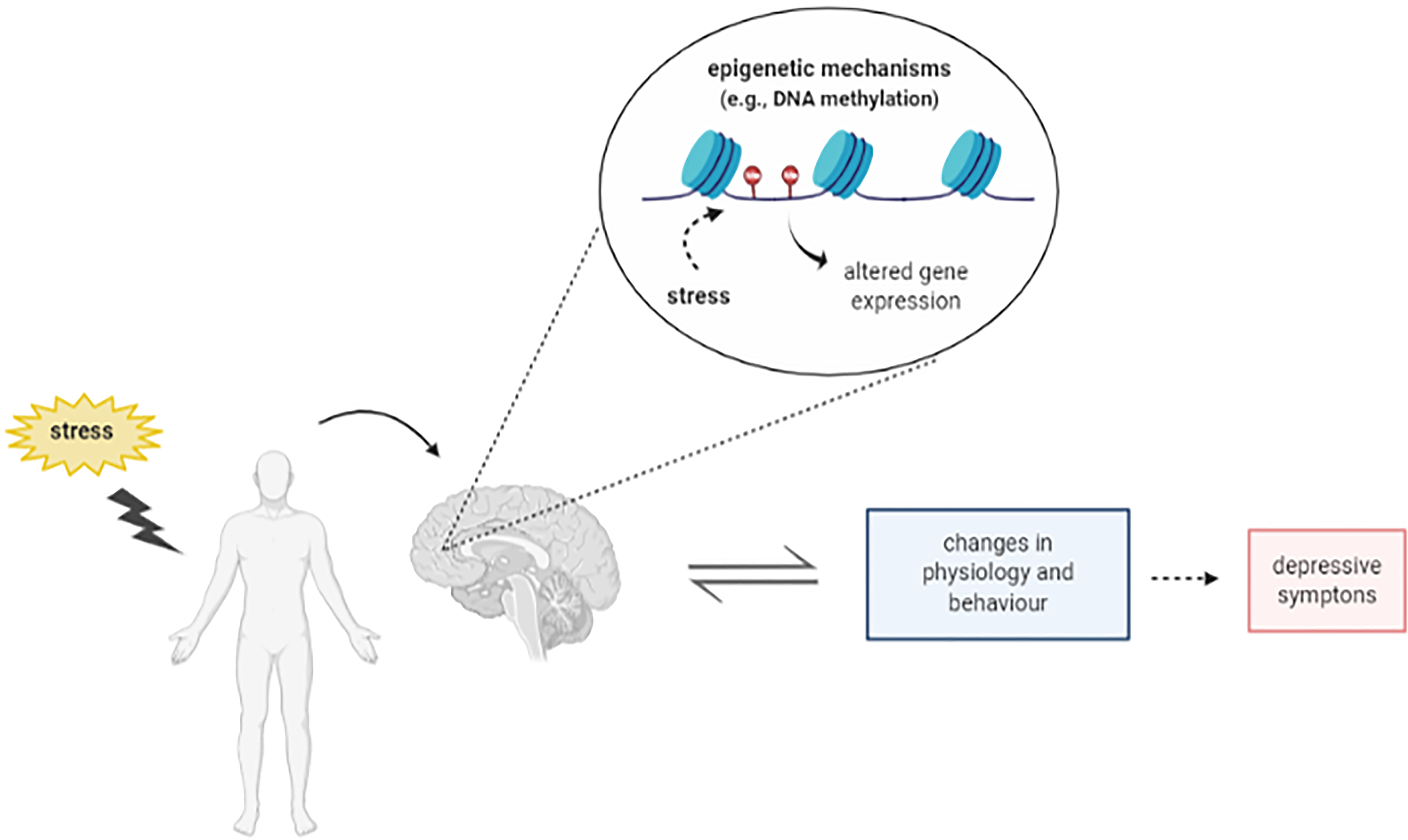
Figure 1. Schematic representation depicting the relation between stress, epigenetic mechanisms and depression. Figure designed using imagens from BioRender.com.
This review summarises our current knowledge on the links between DNAm, stress, and depression. It also discusses the current limitations to better understand these relationships and point to the pharmacological regulation of DNAm as a possible alternative target for the development of novel antidepressant medication.
Stress response and depression
Influence of environmental factors in depression
MDD is a complex, debilitating, and prevalent psychiatric disorder with a set of diverse symptoms including depressed mood, hopelessness, worthlessness, loss of interest in normally pleasurable activities (anhedonia), sleep or eating disturbances, low energy, reduced concentration, impaired cognition, excessive feeling of guilt or loss, and even suicidal thoughts, for at least two weeks (American Psychiatric Association, 2013, Schulz and Arora, Reference Schulz and Arora2015, Otte et al., Reference Otte, Gold, Penninx, Pariante, Etkin, Fava, Mohr and Schatzberg2016).
Depression affects on average 20% of the global population (Vigo et al., Reference Vigo, Thornicroft and Atun2016) and is associated to high levels of morbidity and mortality (Fredman et al., Reference Fredman, Weissman, Leaf and Bruce1988, Bijl and Ravelli, Reference Bijl and Ravelli2000, Kessler et al., Reference Kessler, Akiskal, Ames, Birnbaum, Greenberg, Hirschfeld, Jin, Merikangas, Simon and Wang2006, Kessler and Bromet, Reference Kessler and Bromet2013, Chirita et al., Reference Chirita, Gheorman, Bondari and Rogoveanu2015, Laursen et al., Reference Laursen, Musliner, Benros, Vestergaard and Munk-Olsen2016). It estimates that by 2030 depression will be the leading cause of disability in developed nations (WHO, 2017; 2018). However, its aetiology is still not fully understood.
Genetic factors increase in around 30% the risk of depression (Kendler and Gardner, Reference Kendler and Gardner2016, Smoller, Reference Smoller2016, Shadrina et al., Reference Shadrina, Bondarenko and Slominsky2018, Pettersson et al., Reference Pettersson, Lichtenstein, Larsson, Song, Agrawal, Borglum, Bulik, Daly, Davis, Demontis, Edenberg, Grove, Gelernter, Neale, Pardinas, Stahl, Walters, Walters, Sullivan, Posthuma and Polderman2019). Epidemiologic studies have identified the important genetic contribution to MDD, expressed by the high concordance between monozygotic twins (Sullivan et al., Reference Sullivan, Neale and Kendler2000), and polymorphisms that increase the vulnerability to the development of this disorder (Fabbri et al., Reference Fabbri, Di Girolamo and Serretti2013).
Several lines of evidence indicate that genes are modulated by environmental factors, especially exposure to stress (Higuchi et al., Reference Higuchi, Uchida, Yamagata, Otsuki, Hobara, Abe, Shibata and Watanabe2011). Of note, studies have shown that the first episode of depression is preceded by a stressful event in about 60% of the cases (Post and Silberstein, Reference Post and Silberstein1994). In addition, a higher prevalence of stressful episodes is observed in the life of depressed compared to healthy individuals (Peyrot et al., Reference Peyrot, Middeldorp, Jansen, Smit, De Geus, Hottenga, Willemsen, Vink, Virding, Barragan, Ingelman-Sundberg, Sim, Boomsma and Penninx2013, Klengel and Binder, Reference Klengel and Binder2015, Lopizzo et al., Reference Lopizzo, Bocchio Chiavetto, Cattane, Plazzotta, Tarazi, Pariante, Riva and Cattaneo2015, Richter-Levin and Xu, Reference Richter-Levin and Xu2018).
Although it has been possible to treat depression for decades, depressive symptoms are only reversed after chronic treatment (2–4 weeks) with the currently available antidepressant drugs. However, about 30% of the patients treated with conventional antidepressants do not respond to any medication (Berton and Nestler, Reference Berton and Nestler2006, Johnston et al., Reference Johnston, Powell, Anderson, Szabo and Cline2019). Even among those responsive, about 2/3 do not present complete remission (Rosenzweig-Lipson et al., Reference Rosenzweig-Lipson, Beyer, Hughes, Khawaja, Rajarao, Malberg, Rahman, Ring and Schechter2007, Shelton et al., Reference Shelton, Osuntokun, Heinloth and Corya2010). Overall, these data highlight the need for a better understanding of the neurobiology of depression and to develop faster and more effective treatments, reducing associated health costs, and patient suffering.
Neurobiology of depression and antidepressant action
The discovery that the first antidepressants act by blocking noradrenaline or serotonin reuptake led to the emergence of the monoaminergic theory of depression (Schildkraut, Reference Schildkraut1965, Coppen, Reference Coppen1972, Schildkraut, Reference Schildkraut1995, Castren, Reference Castren2005). This theory postulated that monoaminergic neurotransmission is impaired in depressed individuals, and it is restored after chronic treatment with antidepressants. Even today, most of the antidepressants clinically used, such as tricyclic and selective serotonin reuptake inhibitors, facilitate this neurotransmission. However, the monoaminergic theory is overly simplistic since it does not explain the latency period for the therapeutic response (Castren, Reference Castren2005, Papakostas and Ionescu, Reference Papakostas and Ionescu2015, Otte et al., Reference Otte, Gold, Penninx, Pariante, Etkin, Fava, Mohr and Schatzberg2016, Ferrari and Villa, Reference Ferrari and Villa2017).
To explain this latency, the molecular or neurotrophic hypothesis of depression assumes that the stress triggers intracellular signalling cascades that would result in reduced expression of genes important for plasticity in limbic structures, such as BDNF(Duman et al., Reference Duman, Heninger and Nestler1997, Agid et al., Reference Agid, Buzsaki, Diamond, Frackowiak, Giedd, Girault, Grace, Lambert, Manji, Mayberg, Popoli, Prochiantz, Richter-Levin, Somogyi, Spedding, Svenningsson and Weinberger2007). Chronic administration of antidepressant drugs in stressed animals facilitates monoaminergic signalling, restores neurogenesis, and increases dendritic arborisation in the hippocampus and prefrontal cortex (Malberg and Duman, Reference Malberg and Duman2003, Rodrigues et al., Reference Rodrigues, Ledoux and Sapolsky2009, Castren and Rantamaki, Reference Castren and Rantamaki2010, Kimpton, Reference Kimpton2012, Liu et al., Reference Liu, Ge, Leng, Pan, Fan, Yang and Cui2017). Therefore, gene expression regulation has been proposed as an important molecular mechanism mediating the long-term adaptations related to stress, depression, and antidepressant effect (Tsankova et al., Reference Tsankova, Renthal, Kumar and Nestler2007, Krishnan and Nestler, Reference Krishnan and Nestler2008, Harmer et al., Reference Harmer, Duman and Cowen2017).
The recent discovery of fast-acting antidepressants such as ketamine, an N-methyl-D-aspartate (NMDA) receptor antagonist, has renewed the hope in more rapid and effective therapies (Cipriani et al., Reference Cipriani, Furukawa, Salanti, Chaimani, Atkinson, Ogawa, Leucht, Ruhe, Turner, Higgins, Egger, Takeshima, Hayasaka, Imai, Shinohara, Tajika, Ioannidis and Geddes2018). The exact mechanisms behind ketamine effect are not fully understood, but evidence suggests that it activates the mechanistic target of rapamycin (mTOR) and BDNF signalling, increasing the expression of synaptic proteins (e.g. postsynaptic density protein-95, PSD-95, and synapsin, Syn) in the hippocampus and prefrontal cortex (Gerhard and Duman, Reference Gerhard and Duman2018). These changes would result in a rapid, robust, and sustained antidepressant effects (Price et al., Reference Price, Nock, Charney and Mathew2009, DiazGranados et al., Reference Diazgranados, Ibrahim, Brutsche, Ameli, Henter, Luckenbaugh, Machado-Vieira and Zarate2010, Li et al., Reference Li, Lee, Liu, Banasr, Dwyer, Iwata, Li, Aghajanian and Duman2010, Li et al., Reference Li, Liu, Dwyer, Banasr, Lee, Son, Li, Aghajanian and Duman2011b, Duman and Voleti, Reference Duman and Voleti2012, Zhou et al., Reference Zhou, Wang, Yang, Li, Zhou and Yang2014, Pazini et al., Reference Pazini, Cunha, Rosa, Colla, Lieberknecht, Oliveira and Rodrigues2016, Zhang et al., Reference Zhang, Wang, Lv, Liu, Sun and Tian2018, Abdallah et al., Reference Abdallah, Roache, Averill, Young-Mccaughan, Martini, Gueorguieva, Amoroso, Southwick, Guthmiller, Lopez-Roca, Lautenschlager, Mintz, Litz, Williamson, Keane, Peterson and Krystal2019). Currently, a great body of studies has investigated other compounds that might share similar fast-acting antidepressant action, including glutamatergic neuromodulators (riluzole, agmatine) and glycine-binding site ligands (GLYX-13 and rapastinel) (Zomkowski et al., Reference Zomkowski, Hammes, Lin, Calixto, Santos and Rodrigues2002, Neis et al., Reference Neis, Bettio, Moretti, Rosa, Ribeiro, Freitas, Goncalves, Leal and Rodrigues2016a, Neis et al., Reference Neis, Moretti, Bettio, Ribeiro, Rosa, Goncalves, Lopes, Leal and Rodrigues2016b, Chen et al., Reference Chen, Almeida, Fiori and Turecki2018, Neis et al., Reference Neis, Bettio, Moretti, Rosa, Olescowicz, Fraga, Goncalves, Freitas, Heinrich, Lopes, Leal and Rodrigues2018, Kadriu et al., Reference Kadriu, Musazzi, Henter, Graves, Popoli and Zarate2019).
Brain areas related to depression
Several brain structures have been associated with stress circuits and the therapeutic action of antidepressants, including the amygdala, hypothalamus, ventral tegmental area, nucleus accumbens, hippocampus, prefrontal cortex, among others (Nestler et al., Reference Nestler, Barrot, Dileone, Eisch, Gold and Monteggia2002, Berton and Nestler, Reference Berton and Nestler2006, Krishnan and Nestler, Reference Krishnan and Nestler2008, Price and Drevets, Reference Price and Drevets2010). Plastic, neurochemical, and functional changes in these structures have been associated with the neurobiology of depression (Andrade and Rao, Reference Andrade and Rao2010).
Stress and MDD are associated with hyperactivation of the hypothalamic–pituitary–adrenal (HPA) axis and consequent release of corticotropin-releasing factor (CRF) from paraventricular neurons (PVN) of the hypothalamus, triggering the secretion of adrenocorticotropic hormone (ACTH) from the anterior pituitary and leading to the releasing of glucocorticoids (cortisol in human and corticosterone in rodent) from the adrenal cortex into the blood. Glucocorticoids, through the glucocorticoid receptors (GRs), mediate negative feedback that regulates the HPA axis and stress endocrine responses (Heim and Binder, Reference Heim and Binder2012). They influence the metabolic, physiological, and immunological functions regulated by several stress-associated brain structures such as the hypothalamus, amygdala, prefrontal cortex, and hippocampus (Gillespie and Nemeroff, Reference Gillespie and Nemeroff2005, Pariante and Lightman, Reference Pariante and Lightman2008, Ulrich-Lai and Herman, Reference Ulrich-Lai and Herman2009, Bonfiglio et al., Reference Bonfiglio, Inda, Refojo, Holsboer, Arzt and Silberstein2011, Belda et al., Reference Belda, Fuentes, Daviu, Nadal and Armario2015).
The amygdala is a limbic structure responsible for the regulation of emotions, behaviour, responses to stress, and memory formation (Bhatnagar et al., Reference Bhatnagar, Vining and Denski2004, Li et al., Reference Li, Pleil, Stamatakis, Busan, Vong, Lowell, Stuber and Kash2012, Gilpin et al., Reference Gilpin, Herman and Roberto2015). MDD patients with low serotonin transporter binding potential present hyperactivation of the amygdala (Drevets, Reference Drevets2001, Sheline et al., Reference Sheline, Barch, Donnelly, Ollinger, Snyder and Mintun2001, Siegle et al., Reference Siegle, Steinhauer, Thase, Stenger and Carter2002, Parsey et al., Reference Parsey, Hastings, Oquendo, Huang, Simpson, Arcement, Huang, Ogden, Van Heertum, Arango and Mann2006). It is intimately connected to other brain regions involved in depression such as hippocampus and cortices (Amaral and Insausti, Reference Amaral and Insausti1992). Moreover, chronic mild stress increases the dendritic length and neurite density in this structure (Vyas et al., Reference Vyas, Mitra, Shankaranarayana Rao and Chattarji2002, Khan et al., Reference Khan, Chuhutin, Wiborg, Kroenke, Nyengaard, Hansen and Jespersen2016a,b).
The hippocampus is a subcortical limbic brain structure, closely related to learning, memory, adaptation to stress, and depression. Depressed patients present reduced hippocampal volume (Sheline et al., Reference Sheline, Gado and Kraemer2003, Lorenzetti et al., Reference Lorenzetti, Allen, Fornito and Yucel2009). In animal models, exposure to inescapable and chronic stress causes several hippocampal alterations such as a decrease in number and length of dendritic spines, synaptic loss, and impaired adult neurogenesis (Pittenger and Duman, Reference Pittenger and Duman2008, Serafini, Reference Serafini2012), suggesting a decreased connectivity in this structure (Andrade and Rao, Reference Andrade and Rao2010). These changes are attenuated by chronic antidepressant treatment (Castren and Rantamaki, Reference Castren and Rantamaki2010, Kimpton, Reference Kimpton2012). Recent studies suggest that the dorsal and ventral portions of hippocampus are molecularly and functionally distinct (Leonardo et al., Reference Leonardo, Richardson-Jones, Sibille, Kottman and Hen2006, Fanselow and Dong, Reference Fanselow and Dong2010, Tanti and Belzung, Reference Tanti and Belzung2013, Grigoryan and Segal, Reference Grigoryan and Segal2016, Diniz et al., Reference Diniz, Casarotto, Resstel and Joca2018). This difference should be considered when investigating the role of the hippocampus in stress and depression.
Another structure closely related to the neurobiology of depression is the prefrontal cortex. The prefrontal cortex plays an important role in cognition, learning, memory, decision making, and modulation of behavioural, autonomic, and endocrine in stress responses (Barbas, Reference Barbas1995, Liston et al., Reference Liston, Miller, Goldwater, Radley, Rocher, Hof, Morrison and Mcewen2006, Quirk et al., Reference Quirk, Garcia and Gonzalez-Lima2006, Resstel and Correa, Reference Resstel and Correa2006, Resstel et al., Reference Resstel, Joca, Guimaraes and Correa2006, Girotti et al., Reference Girotti, Adler, Bulin, Fucich, Paredes and Morilak2018). Dysfunctions in this structure are related to the development of mental disorders associated with stress, such as depression and posttraumatic stress disorder – PTSD (Geuze et al., Reference Geuze, Westenberg, Heinecke, De Kloet, Goebel and Vermetten2008, Pereira et al., Reference Pereira, Casarotto, Hiroaki-Sato, Sartim, Guimaraes and Joca2013). Similar to the hippocampus, stressful insults result in plastic modifications in the prefrontal cortex such as apical dendritic atrophy and reductions of synaptogenesis and cortical volume. Antidepressant drugs reverse these morphological changes (Duncan et al., Reference Duncan, Knapp, Johnson and Breese1996, Cook and Wellman, Reference Cook and Wellman2004, Rocher et al., Reference Rocher, Spedding, Munoz and Jay2004, Brown et al., Reference Brown, Henning and Wellman2005, Czeh et al., Reference Czeh, Perez-Cruz, Fuchs and Flugge2008). In line with these data, depressed patients present functional and volume cortical changes, which are sensitive to chronic antidepressant treatment (Harmer et al., Reference Harmer, Mackay, Reid, Cowen and Goodwin2006, Fitzgerald et al., Reference Fitzgerald, Laird, Maller and Daskalakis2008, Koenigs et al., Reference Koenigs, Huey, Calamia, Raymont, Tranel and Grafman2008, Yoshimura et al., Reference Yoshimura, Okamoto, Onoda, Matsunaga, Ueda, Suzuki and Shigetoyamawaki2010). In addition, the antidepressant effect of fast-acting drugs, such as ketamine, is associated with neuroplastic changes in the prefrontal cortex such as the rapid recovery of dendritic arborisation and synaptogenesis (Dwyer and Duman, Reference Dwyer and Duman2013, Abdallah et al., Reference Abdallah, Adams, Kelmendi, Esterlis, Sanacora and Krystal2016).
Recently, the lateral habenula nucleus has attracted interest as a relevant brain structure that is also associated with stress coping responses. Stressors led to hyperactivation of neurons in this structure following by negative effects on serotonergic function and an imbalance between glutamatergic and GABAergic signalling (Shabel et al., Reference Shabel, Proulx, Piriz and Malinow2014, Metzger et al., Reference Metzger, Bueno and Lima2017, Tchenio et al., Reference Tchenio, Lecca, Valentinova and Mameli2017, Wang et al., Reference Wang, Li, Feng, Guo, Zhou and Luo2017). These changes contribute to depressive-like behaviours (Aizawa et al., Reference Aizawa, Cui, Tanaka and Okamoto2013, Li et al., Reference Li, Zhou, Liao, Yang, Wong, Henn, Malinow, Yates and Hu2013, Cui et al., Reference Cui, Mizukami, Yanagisawa, Aida, Nomura, Isomura, Takayanagi, Ozawa, Tanaka and Aizawa2014). The deep brain stimulation of the lateral habenula nucleus prevents depressive-like behaviour in animals (Li et al., Reference Li, Piriz, Mirrione, Chung, Proulx, Schulz, Henn and Malinow2011a) and alleviates depressive symptoms in patients (Sartorius et al., Reference Sartorius, Kiening, Kirsch, Von Gall, Haberkorn, Unterberg, Henn and Meyer-Lindenberg2010).
Epigenetic
Epigenetic overview
The human genome encompasses about 20,000 genes containing essential information on the normal growth, development, and survival of the organism. The long DNA strand (2 m) is packed in the compact nucleus of eukaryotic cells (10 μm in diameter). This process depends on the association of the DNA with histone and non-histone proteins resulting in a highly complex and organised structure called chromatin. The fundamental and functional unit of chromatin is the nucleosome consisting of a 146 base pairs (bp) envelope of DNA surrounded by an octameric histone complex with four histone proteins (H2A, H2B, H3, and H4). Mechanisms of repair, replication, recombination, transcription, dynamic opening, and closure of the chromatin structure are fundamental for homoeostasis (Hauer and Gasser, Reference Hauer and Gasser2017).
In the 1940s, Conrad Hal Waddington, a leading embryologist and geneticist, sought to explain how different cell types could be generated from cells containing the same common genome, thus generating different phenotypes that would result from the interaction between both genes and the environment. He believed that genes are important in determining the development of the organism, but environmental-induced modifications could occur and alter this development. He called this process causal embryology or “epigenetic” (Slack, Reference Slack2002). The definition of epigenetics, a word that means “above the genome”, has been modified over the years. It is now broadly understood as the process by which environmental factors can lead to stable changes in chromatin structure, altering gene expression but changing the primary sequence of bases in the DNA (Holliday, Reference Holliday2006, Allis and Jenuwein, Reference Allis and Jenuwein2016, Gayon, Reference Gayon2016). Epigenetic alterations have been related to the neurobiology of diverse illnesses, including psychiatric disorders (Tsankova et al., Reference Tsankova, Renthal, Kumar and Nestler2007, Mitchelmore and Gede, Reference Mitchelmore and Gede2014, Cattaneo et al., Reference Cattaneo, Macchi, Plazzotta, Veronica, Bocchio-Chiavetto, Riva and Pariante2015, Bartlett et al., Reference Bartlett, Singh and Hunter2017, Uchida et al., Reference Uchida, Yamagata, Seki and Watanabe2018, Bhandari et al., Reference Bhandari, Paliwal and Kuhad2020, Forero and Gonzalez-Giraldo, Reference Forero and Gonzalez-Giraldo2020, Jackson, Reference Jackson2020).
The biological properties of chromatin have been extensively studied since the 19th century. In 1884, Albrecht Kossel identified the histones and determined that these basic proteins were associated with DNA. Thanks to this pivotal contribution, he received the Nobel Prize in Physiology and Medicine in 1910. Since then, a large number of studies have investigated the structure and function of the histones (Grayson and Guidotti, Reference Grayson and Guidotti2013). Transitions between euchromatin and heterochromatin are associated with active or inactive transcription, respectively, and are mediated by modifications in the structure of the histones that constitute the nucleosome (Jenuwein and Allis, Reference Jenuwein and Allis2001).
Histone modifications occur mainly in histones 3 (H3) and 4 (H4) and comprise phosphorylation, ubiquitination, acetylation and deacetylation, and methylation and demethylation (Mersfelder and Parthun, Reference Mersfelder and Parthun2006, Bannister and Kouzarides, Reference Bannister and Kouzarides2011). Regarding the amino acids present along the histone tails, lysine (K) and arginine (R) are preferentially subject to methylation (Jenuwein and Allis, Reference Jenuwein and Allis2001). Histones are acetylated in K-residues on the N-terminal branching as part of the genetic regulation, being catalysed by the histone acetyltransferases (HATs) enzymes (Fig. 2). In deacetylation, in turn, acetylated histones lose the acetyl group by the action of histone deacetylase enzymes (HDACs, (Grayson and Guidotti, Reference Grayson and Guidotti2013). Histone acetylation is primarily associated with decondensed chromatin followed by increased accessibility of DNA to transcriptional factors and gene activation, being able to facilitate the expression of genes important for cellular plasticity and involved with the neurobiology of depression and antidepressants effects (Strekalova et al., Reference Strekalova, Spanagel, Bartsch, Henn and Gass2004, Krishnan and Nestler, Reference Krishnan and Nestler2008).
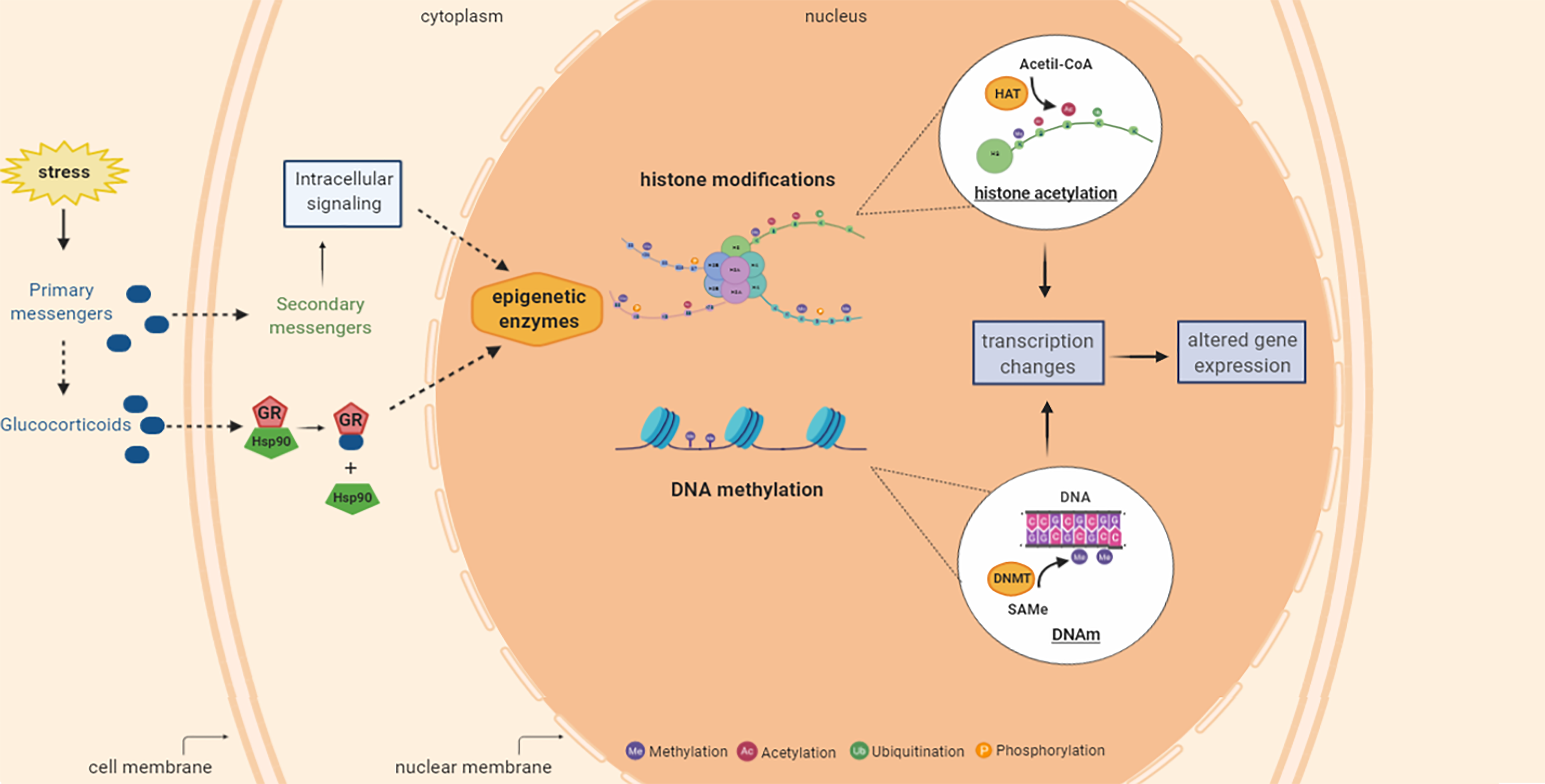
Figure 2. Summary of stress-induced epigenetic changes including histone acetylation and DNA methylation processes that can occur in mammalian tissues including brain. Stress is related with increased glucocorticoid levels and activation of signalling cascades that can result in the epigenetic enzymes modulation (activation or inhibition followed by alterations in the epigenetic mechanisms and transcription gene. Histone modifications can add various groups (methyl, acetyl, phosphoryl and ubiquitin) to the tails of the histone proteins. The histone acetyl transferase (HAT) enzyme catalyses the transfer of the acetyl group from acetyl-CoA to the histone protein. DNA methylation (DNAm) is the addition of methyl group from S-adenosyl-L-methionine (SAMe), catalysed by DNA methyltransferase (DNMT) enzymes, to the cytosine resulting in 5-methyl cytosine. These epigenetic mechanisms can affect the gene transcription and protein expression. H, histone protein; Acetyl-CoA, acetyl coenzyme A; HAT, histone acetyltransferase; SAMe, S-adenosyl-L-methionine; DNMT, DNA methyltransferase; DNAm, DNA methylation. Figure designed using imagens from BioRender.com.
In addition to post-translational modifications in histone tails (e.g., methylation, acetylation, phosphorylation, and ubiquitination), epigenetic mechanisms encompass covalent modifications (e.g., DNAm, detailed below) and nontranslational mechanisms of gene modulation (e.g., microRNAs, miRNAs, ribonucleic acid) (Issler and Chen, Reference Issler and Chen2015, Luoni and Riva, Reference Luoni and Riva2016, Ivanova et al., Reference Ivanova, Bozhilova, Kaneva and Milanova2018). These mechanisms have been extensively discussed elsewhere (O’Carroll and Schaefer, Reference O’carroll and Schaefer2013, Correia de Sousa et al., Reference Correia De Sousa, Gjorgjieva, Dolicka, Sobolewski and Foti2019, Tolsma and Hansen, Reference Tolsma and Hansen2019) and are not the scope of the current review.
Epigenetic mechanisms could promote long-lasting effects on mature neurons, interfering with complex neural mechanisms such as plasticity synaptic and neurogenesis (Tsankova et al., Reference Tsankova, Renthal, Kumar and Nestler2007, Karpova et al., Reference Karpova, Sales and Joca2017). The increase or decrease of chromatin condensation makes it more difficult or easier, respectively, the access of the transcriptional machinery to specific promoter regions, resulting in messenger RNA (mRNA) and protein levels changes (Tsankova et al., Reference Tsankova, Berton, Renthal, Kumar, Neve and Nestler2006, Tsankova et al., Reference Tsankova, Renthal, Kumar and Nestler2007, Krishnan and Nestler, Reference Krishnan and Nestler2008).
Although less understood, epigenetic mechanisms have recently been proposed to also play a crucial role in the rapid stress coping responses (Chandramohan et al., Reference Chandramohan, Droste, Arthur and Reul2008, Saunderson et al., Reference Saunderson, Spiers, Mifsud, Gutierrez-Mecinas, Trollope, Shaikh, Mill and Reul2016, Mifsud et al., Reference Mifsud, Saunderson, Spiers, Carter, Trollope, Mill and Reul2017) (Fig. 2). For example, stress and antidepressants modify cochaperones (mainly FK506-binding protein 51 and 52, FKBP51 and 52), which proteins that participate in the function of other chaperone proteins that act facilitating the folding proteins (Saibil, Reference Saibil2013). FKBP51 and 52 modulate the phosphorylation and activity of DNA methyltransferase (DNMT, an enzyme that catalyses DNAm), altering the DNAm levels (Gassen et al., Reference Gassen, Fries, Zannas, Hartmann, Zschocke, Hafner, Carrillo-Roa, Steinbacher, Preissinger, Hoeijmakers, Knop, Weber, Kloiber, Lucae, Chrousos, Carell, Ising, Binder, Schmidt, Ruegg and Rein2015). Still, the exposure to acute stressful events results in DNA demethylation of c-Fos (FBJ murine osteosarcoma viral oncogene homolog), an immediate-early gene, leading to its increased expression in the dentate gyrus of the hippocampus (Saunderson et al., Reference Saunderson, Spiers, Mifsud, Gutierrez-Mecinas, Trollope, Shaikh, Mill and Reul2016).
DNA methylation
DNAm usually occurs by a covalent attachment of a methyl group from the S-adenosyl-L-methionine (SAMe) onto the C5 position of cytosine residue of DNA, primarily occurring on cytosine-phosphate-guanine (CpG) dinucleotides clusters, resulting in 5-methyl cytosine (5mC) (Fig. 3). While showing cell- and tissue-specific differences, in mammalian cells DNAm often occurs in cytosines located on the so-named CpGs islands (CGIs, regions with a high frequency of CpG sequences). These islands are typically found in or near promoter regions resulting in 70–80% of methylated CpGs during DNAm (Moore et al., Reference Moore, Le and Fan2013, Ziller et al., Reference Ziller, Gu, Muller, Donaghey, Tsai, Kohlbacher, De Jager, Rosen, Bennett, Bernstein, Gnirke and Meissner2013, Li and Zhang, Reference Li and Zhang2014).
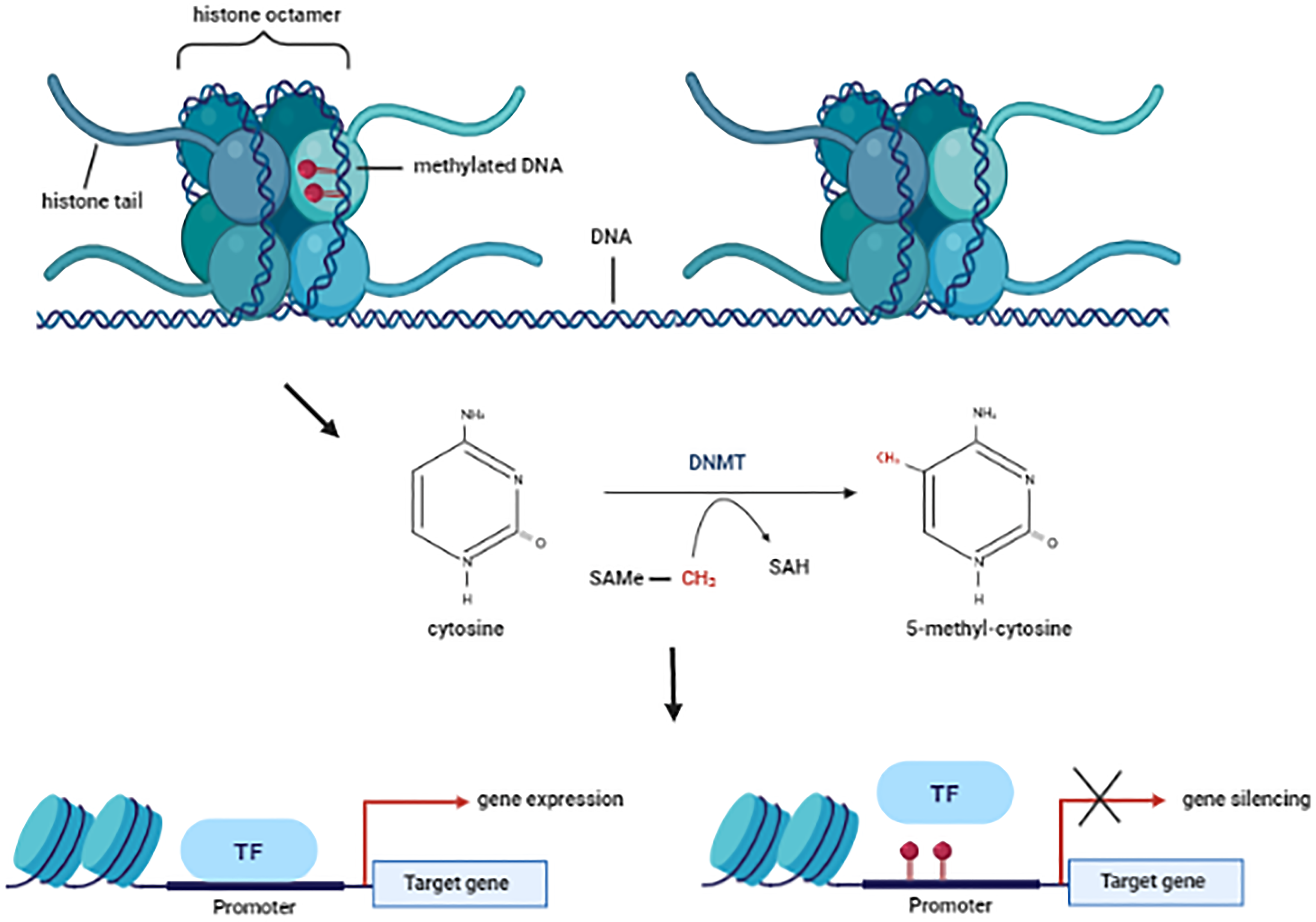
Figure 3. Schematic chromatin structure and DNA methylation mechanism. DNMTs catalyse the transfer of methyl group from S-adenosyl-L-methionine (SAMe) to the C5 position of the cytosine residue resulting in the S-adenosyl-L-homocysteine (SAH) and 5-methyl cytosine (5mC). This covalent modification is associated with low acessibily of the transcrition factors (TF) to the promoter gene following by gene silencing. SAMe, S-adenosyl-L-methionine; DNMT, DNA methyltransferase; SAH, S-adenosyl-L-homocysteine; TF, transcription fator. Figure designed using imagens from BioRender.com.
Classically, DNAm in promoters containing CGIs is associated with transcriptional reduction (Antequera, Reference Antequera2003, Lorincz et al., Reference Lorincz, Dickerson, Schmitt and Groudine2004, Brenet et al., Reference Brenet, Moh, Funk, Feierstein, Viale, Socci and Scandura2011). The methyl-CpG-binding domain (MBD) proteins bind preferentially to methylated CGIs. In addition, MBD proteins also form a complex with corepressor and chromatin-modifying proteins, resulting in gene silencing (Wajed et al., Reference Wajed, Laird and Demeester2001, Jones, Reference Jones2012). Moreover, the methyl binding can also mechanically block the access of transcription factors to promoter regions, also resulting in transcriptional repression followed by gene inactivation (Hark et al., Reference Hark, Schoenherr, Katz, Ingram, Levorse and Tilghman2000). Contrary to this, promoter hypermethylation has also been associated with increased transcriptional activity (reviewed in Smith et al., Reference Smith, Sen, Weeks, Eccles and Chatterjee2020), showing that the DNAm-mediated gene regulation is a very complex and unclear mechanism. It is possible that the DNAm may block the interaction between DNA and transcriptional repressors facilitating gene transcription (Nabilsi et al., Reference Nabilsi, Broaddus and Loose2009, Bahar Halpern et al., Reference Bahar Halpern, Vana and Walker2014, Jia et al., Reference Jia, Wang, Li, Tao, Chang, Hua, Yu, Wong and Feng2016). However, further studies are required for a better understanding of this mechanism.
Although DNAm occurs primarily in cytosine-guanine (CpG) dinucleotides, methylation also occurs in non-CpG sites, particularly in neuronal cells. The functions of these processes are unclear (Lister et al., Reference Lister, Mukamel, Nery, Urich, Puddifoot, Johnson, Lucero, Huang, Dwork, Schultz, Yu, Tonti-Filippini, Heyn, Hu, Wu, Rao, Esteller, He, Haghighi, Sejnowski, Behrens and Ecker2013). Additionally, DNAm in non-promoter regions has variable and unpredictable effects on gene expression (Antequera, Reference Antequera2003, Lorincz et al., Reference Lorincz, Dickerson, Schmitt and Groudine2004, Hellman and Chess, Reference Hellman and Chess2007, Ball et al., Reference Ball, Li, Gao, Lee, Leproust, Park, Xie, Daley and Church2009, Aran et al., Reference Aran, Toperoff, Rosenberg and Hellman2011, Jones, Reference Jones2012, Thomas et al., Reference Thomas, Sai and Wells2012, Kulis et al., Reference Kulis, Queiros, Beekman and Martin-Subero2013, Moore et al., Reference Moore, Le and Fan2013).
The methylation reaction is catalysed by DNA methyltransferases (DNMTs) enzymes (Fig. 3), detailed below. The first suggestion that DNAm (or demethylation) plays an important biological role was done by Griffith and Mahler, in Reference Griffith and Mahler1969. They proposed that this epigenetic mechanism could be the basis of long-term memory (Griffith and Mahler, Reference Griffith and Mahler1969). In 1975, two other groups suggested that DNAm would change gene expression, resulting in the repression of diverse genes (Holliday and Pugh, Reference Holliday and Pugh1975, Riggs, Reference Riggs1975). These studies also proposed that the methylation pattern was inherited through the action of a maintenance methylase enzyme capable of recognising hemimethylated DNA after replication, but unable to act on the unmethylated DNA.
DNAm has now been implicated in regulating gene activity in the adult brain under both normal and pathological conditions (Feng et al., Reference Feng, Zhou, Campbell, Le, Li, Sweatt, Silva and Fan2010). Intracellular signal transduction events, among other functions, can activate or inhibit transcription factors. The regulation of transcriptional activity by DNA-binding transcription factors depends on the interaction between several of these factors, including co-activators or co-repressors proteins and the underlying structure of the chromatin. The synaptic activity can activate or repress gene expression through chromatin remodeling. This mechanism regulates the expression of genes important for neural activity, survival, morphology, integration, and behaviour regulation (Zuckerkandl, Reference Zuckerkandl1975, Whitehouse et al., Reference Whitehouse, Rando, Delrow and Tsukiyama2007, Sweatt, Reference Sweatt2016, Uchida et al., Reference Uchida, Yamagata, Seki and Watanabe2018).
DNMT enzymes comprise five subtypes (DNMT1, DNMT2, DNMT3a, DNMT3b, and DNMT3L). Three of these subtypes (DNMT1, DNMT3a, and DNMT3b) are functionally active and widely studied. DNMT1 plays a maintenance role by copying the preexisting methylation of the parent into the daughter cell DNA during the replication phase. DNMTs 3a and 3b, in turn, are responsible for the new methylations in unmethylated CpG sequences (Jeltsch, Reference Jeltsch2006). DNMT2 is usually inactive or with low activity in both in vitro and in vivo studies (Herman and Baylin, Reference Herman and Baylin2003, Liu et al., Reference Liu, Wylie, Andrews and Tollefsbol2003). DNMT3L also lacks catalytic activity but is important for methylation by recruiting the active DNMTs (Bourc’his et al., Reference Bourc’his, Xu, Lin, Bollman and Bestor2001, Bourc’his and Bestor, Reference Bourc’his and Bestor2004, Ooi et al., Reference Ooi, Qiu, Bernstein, Li, Jia, Yang, Erdjument-Bromage, Tempst, Lin, Allis, Cheng and Bestor2007, Liao et al., Reference Liao, Karnik, Gu, Ziller, Clement, Tsankov, Akopian, Gifford, Donaghey, Galonska, Pop, Reyon, Tsai, Mallard, Joung, Rinn, Gnirke and Meissner2015, Long et al., Reference Long, Smiraglia and Campbell2017, Hervouet et al., Reference Hervouet, Peixoto, Delage-Mourroux, Boyer-Guittaut and Cartron2018).
During cell proliferation, DNMT1 is located in replication loci and primarily methylates the unmethylated DNA of daughter cells, maintaining the pattern of parental methylation across generations. In divided cells, DNMT1 is concentrated in replication sites during the S phase in order to maintain DNAm profile after the DNA synthesis of the daughter cell. DNMT1 deletion in progenitor neural cells results in reduced DNAm levels in post-mitotic neurons (Feng et al., Reference Feng, Zhou, Campbell, Le, Li, Sweatt, Silva and Fan2010, Hervouet et al., Reference Hervouet, Peixoto, Delage-Mourroux, Boyer-Guittaut and Cartron2018). However, DNMT1 participates not only in maintenance but also in new methylations (Pradhan et al., Reference Pradhan, Bacolla, Wells and Roberts1999, Gowher et al., Reference Gowher, Stockdale, Goyal, Ferreira, Owen-Hughes and Jeltsch2005, Hervouet et al., Reference Hervouet, Peixoto, Delage-Mourroux, Boyer-Guittaut and Cartron2018).
DNMTs 3a and 3b (known as “de novo” DNMTs) are more actively involved in the methylation of unmethylated DNA, establishing new methylation patterns (Feng et al., Reference Feng, Zhou, Campbell, Le, Li, Sweatt, Silva and Fan2010, Hervouet et al., Reference Hervouet, Peixoto, Delage-Mourroux, Boyer-Guittaut and Cartron2018). DNMT 3b is widely expressed in the early stages of neurogenesis (Okano et al., Reference Okano, Bell, Haber and Li1999) but is also present in adults (Feng et al., Reference Feng, Chang, Li and Fan2005, Feng et al., Reference Feng, Zhou, Campbell, Le, Li, Sweatt, Silva and Fan2010, Hervouet et al., Reference Hervouet, Peixoto, Delage-Mourroux, Boyer-Guittaut and Cartron2018). DNMT 3a is present in both the mature and development brain, suggesting its involvement in embryonic development and adult neuronal function. The two enzymes are often co-localized and associated with heterochromatin regions independent of the cell cycle (Bachman et al., Reference Bachman, Rountree and Baylin2001), possibly maintaining DNAm in pre- and post-mitotic cells (Feng et al., Reference Feng, Zhou, Campbell, Le, Li, Sweatt, Silva and Fan2010, Hervouet et al., Reference Hervouet, Peixoto, Delage-Mourroux, Boyer-Guittaut and Cartron2018).
Pharmacological manipulation of DNA methylation
In the 1960s, Sorm et al. (Reference Sorm, Piskala, Cihak and Vesely1964) synthesised the first epigenetic drugs (or epi-drugs) with possible inhibitory action on DNMTs, 5-azacytidine (5-AzaC), and 5-Aza-2′-deoxycytidine (5-AzaD or decitabine; Sorm et al., Reference Sorm, Piskala, Cihak and Vesely1964). Initially, these drugs were tested as antimetabolic nucleoside inhibitors for the treatment of acute myeloid leukemia. In 1968, Sorm and Veseley demonstrated that they cause a potent antileukemic effect in mice (Sorm and Vesely, Reference Sorm and Vesely1968). The clinical studies began in the 1980s (Rivard et al., Reference Rivard, Momparler, Demers, Benoit, Raymond, Lin and Momparler1981, Momparler et al., Reference Momparler, Rivard and Gyger1985) and, corroborating the preclinical findings, indicated that the 5-AzaD treatment induces complete remission in leukemic patients (Richel et al., Reference Richel, Colly, Kluin-Nelemans and Willemze1991). In 2004, the Food and Drug Administration (FDA) approved the first DNMT inhibitor (DNMTi) drug, 5-AzaC, and in 2006 the second, 5-AzaD, for clinical use in myelodysplastic syndromes. Their mechanisms are not fully understood but it is known that they inhibit DNAm and, at high doses, can be cytotoxic.
Aberrant promoter DNAm in tumour suppressor genes inhibits their expression and can contribute to tumourigenesis. The reactivation of these genes by inhibition of DNAm induced by DNMTi has potential antitumoural effects. Several studies in tumour cells have shown that genes involved in the development and progression of cancer are hypomethylated, whereas those associated with tumour suppression are hypermethylated (Stresemann and Lyko, Reference Stresemann and Lyko2008). DNMTi reversed this methylation pattern by reactivating tumour suppressor genes, such as p15 (Momparler, Reference Momparler2005, Yoo and Jones, Reference Yoo and Jones2006, Karahoca and Momparler, Reference Karahoca and Momparler2013).
DNMTi are divided into two groups, nucleoside and non-nucleoside, which inhibit DNMTs through different mechanisms. Nucleosidic DNMTi, such as 5-AzaC, 5-AzaD, zebularine, SGI-110, and CP-4200, are chemical analogues of cytidine, being integrated into the DNA molecule during replication (S-phase). They covalently bind to the DNMTs, causing an irreversible blockage of these enzymes and preventing DNAm (Stresemann and Lyko, Reference Stresemann and Lyko2008, Diesch et al., Reference Diesch, Zwick, Garz, Palau, Buschbeck and Gotze2016). 5-AzaC and 5-AzaD are administered as prodrugs with low oral bioavailability (Zhang et al., Reference Zhang, Sun, Gao, Jin, Xu, Lian, Sun, Sun, Liu, Fan, Zhang and He2013). They also have a short plasma half-life, approximately 30 minutes after intravenous administration (Daskalakis et al., Reference Daskalakis, Blagitko-Dorfs and Hackanson2010, Estey, Reference Estey2013, Navada et al., Reference Navada, Steinmann, Lubbert and Silverman2014). In general, the cellular uptake of these prodrugs depends on diverse transporters, including nucleoside transporter proteins (SLC28 and SLCA29 gene families; Pastor-Anglada et al., Reference Pastor-Anglada, Molina-Arcas, Casado, Bellosillo, Colomer and Gil2004, Qin et al., Reference Qin, Jelinek, Si, Shu and Issa2009). These drugs are activated by uridine-cytidine kinase and deoxycytidine kinase and are inactivated by deamination (Pastor-Anglada et al., Reference Pastor-Anglada, Molina-Arcas, Casado, Bellosillo, Colomer and Gil2004, Qin et al., Reference Qin, Jelinek, Si, Shu and Issa2009, Daskalakis et al., Reference Daskalakis, Blagitko-Dorfs and Hackanson2010, Valencia et al., Reference Valencia, Masala, Rossi, Martino, Sanna, Buchi, Canzian, Cilloni, Gaidano, Voso, Kosmider, Fontenay, Gozzini, Bosi and Santini2014). Moreover, nucleoside inhibitors are potentially nonspecific cytotoxic compounds with structural instability, which restricts their clinical therapy (Yoo and Jones, Reference Yoo and Jones2006, Stresemann and Lyko, Reference Stresemann and Lyko2008, Gros et al., Reference Gros, Fahy, Halby, Dufau, Erdmann, Gregoire, Ausseil, Vispe and Arimondo2012).
Non-nucleoside inhibitors, such as RG108, hydralazine, procaine, procainamide, IM25, and disulfiram, have a variety of action mechanisms. They are all independent of cell division. Their mechanisms include non-covalent inhibition in the DNA catalytic sites, prevention of their enzymatic activity (Lyko and Brown, Reference Lyko and Brown2005, Mai and Altucci, Reference Mai and Altucci2009), reduction of DNMTs affinity for the DNA (Zambrano et al., Reference Zambrano, Segura-Pacheco, Perez-Cardenas, Cetina, Revilla-Vazquez, Taja-Chayeb, Chavez-Blanco, Angeles, Cabrera, Sandoval, Trejo-Becerril, Chanona-Vilchis and Duenas-Gonzalez2005, Castellano et al., Reference Castellano, Kuck, Sala, Novellino, Lyko and Sbardella2008, Datta et al., Reference Datta, Ghoshal, Denny, Gamage, Brooke, Phiasivongsa, Redkar and Jacob2009), inhibition of methyl donor proteins (Cui et al., Reference Cui, Wakai, Shirai, Yokoyama, Hatakeyama and Hirano2006), or suppression of DNMTs expression (Pina et al., Reference Pina, Gautschi, Wang, Sanders, Schmitz, France, Cornell-Kennon, Sambucetti, Remiszewski, Perez, Bair and Crews2003). Usually, these drugs have a short plasma half-life (approximately 4 h for RG108 after subcutaneous administration) (Schneeberger et al., Reference Schneeberger, Stenzig, Hubner, Schaefer, Reichenspurner and Eschenhagen2016) and reduced adverse effects (Xu et al., Reference Xu, Hu, Luo and Liang2016). Additionally, natural compounds such as curcumin, genistein, EGCG, and resveratrol also act by indirect inhibition of DNMTs (revised in Lascano et al., Reference Lascano, Lopez and Arimondo2018).
DNMTi drugs have a wide distribution in body fluids, crossing the blood-brain barrier (Marcucci et al., Reference Marcucci, Silverman, Eller, Lintz and Beach2005, Schneeberger et al., Reference Schneeberger, Stenzig, Hubner, Schaefer, Reichenspurner and Eschenhagen2016) and possibly reversing situations where there is increased methylation in diverse tissues including CNS.
DNA methylation in stress and depression
Preclinical studies
Preclinical studies have reported that stress modulates DNAm (Tables 1 and 2). Exposure to stress stimuli in the early developmental stages results in persistent epigenetic changes associated with long-lasting gene expression changes and influencing neural and behavioural functions in adulthood (Weaver et al., Reference Weaver, Cervoni, Champagne, D’alessio, Sharma, Seckl, Dymov, Szyf and Meaney2004, Tsankova et al., Reference Tsankova, Berton, Renthal, Kumar, Neve and Nestler2006). Diverse studies show stress-induced hypermethylation and subsequent reduced gene expression (Table 1). Gestational stress increases the expression of DNMT1 and DNAm on the BDNF promoter, resulting in decreased BDNF transcripts and protein levels in the hippocampus of the offspring (Zheng et al., Reference Zheng, Fan, Zhang and Dong2016). Still, male mice of dams prenatally restraint stressed show increased DNAm in the promoter regions of glutamic decarboxylase 67 (Gad67), reelin (Reln), and BDNF (IX) (Dong et al., Reference Dong, Locci, Gatta, Grayson and Guidotti2019). These changes in DNAm profile are associated with their reduced expression. Offspring prenatally exposed to the bisphenol A, considered an endocrine-disrupting chemical associated with long-term behavioural effects (Braun et al., Reference Braun, Kalkbrenner, Calafat, Yolton, Ye, Dietrich and Lanphear2011, Kundakovic and Champagne, Reference Kundakovic and Champagne2011, Perera et al., Reference Perera, Vishnevetsky, Herbstman, Calafat, Xiong, Rauh and Wang2012), shown long-lasting BDNF IV hypermethylation in the hippocampus and blood of mice (Kundakovic et al., Reference Kundakovic, Gudsnuk, Herbstman, Tang, Perera and Champagne2015). Prenatal exposure to maternal depression increases neonatal DNAm of glucocorticoid receptor gene (NR3C1) in cord blood cells in humans (Oberlander et al., Reference Oberlander, Weinberg, Papsdorf, Grunau, Misri and Devlin2008). It also increases DNAm of BDNF IV promoter and decreases its mRNA in the amygdala and hippocampus of rodents (Boersma et al., Reference Boersma, Lee, Cordner, Ewald, Purcell, Moghadam and Tamashiro2014).
Table 1. Studies showing modulation and increased DNA methylation (DNAm) after stress

Note: MeDIP, methylated DNA immunoprecipitation; PCR, polymerase chain reaction; DNAm, DNA methylation; DNMT, DNA methyltransferase; mRNA, messenger RNA; CpG, cytosine-phosphate-guanine; GR, glucocorticoid receptor; ELISA, enzyme-linked immunosorbent assay; CA, cornu ammonis; qPCR, quantitative polymerase chain reaction; PBMCs, peripheral mononuclear blood cells; AMPA, α-amino-3-hydroxy-5-methyl-4-isoxazolepropionic acid.
Table 2. Studies showing no change, decreased DNA methylation (DNAm), and complex gene expression modulation by DNAm after stress
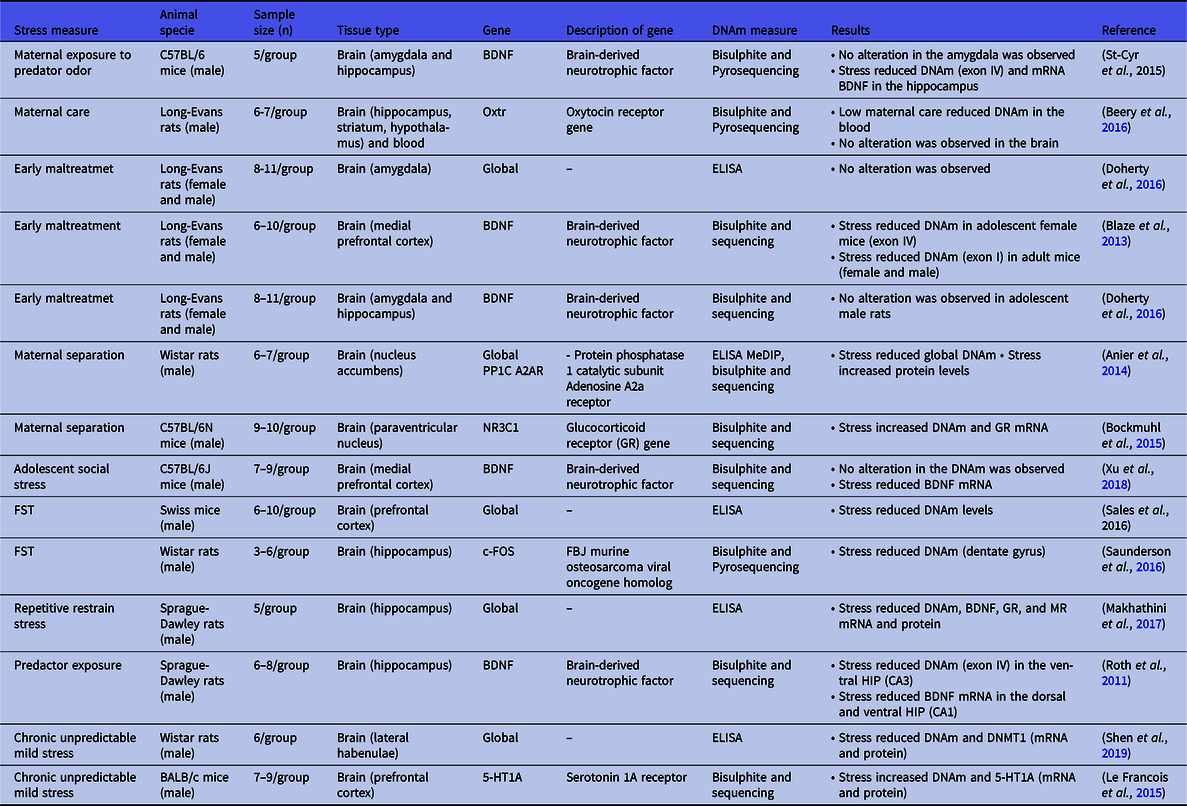
Note: DNAm, DNA methylation; mRNA, messenger RNA; ELISA, enzyme-linked immunosorbent assay; MeDIP, methylated DNA immunoprecipitation; FST, forced swimming test; GR, glucocorticoid receptor; MR, mineralocorticoid receptor; CA, cornu ammonis; DNMT, DNA methyltransferase.
DNAm changes have been associated with maternal care. Low maternal care increased DNAm in the NR3C1 promoter region and decreased its gene expression in the hippocampus (Weaver et al., Reference Weaver, Cervoni, Champagne, D’alessio, Sharma, Seckl, Dymov, Szyf and Meaney2004, Szyf et al., Reference Szyf, Weaver and Meaney2007, Tsankova et al., Reference Tsankova, Renthal, Kumar and Nestler2007). Maternal separation stress was associated with hypermethylation in the rat hippocampus (McCoy et al., Reference Mccoy, Rana, Stringfellow, Day, Wyss, Clinton and Kerman2016). This stressor also increased DNMTs expression (Anier et al., Reference Anier, Malinovskaja, Pruus, Aonurm-Helm, Zharkovsky and Kalda2014) and DNAm in the protein phosphatase 1 catalytic subunit (PP1C), a neuronal plasticity-related gene, in the nucleus accumbens, followed by its transcriptional downregulation (Anier et al., Reference Anier, Malinovskaja, Pruus, Aonurm-Helm, Zharkovsky and Kalda2014). Similarly, DNAm in the nucleus accumbens but not in the prefrontal cortex is increased in the promoter of adenosine A2a receptor (A2AR) (Anier et al., Reference Anier, Malinovskaja, Pruus, Aonurm-Helm, Zharkovsky and Kalda2014), which the upregulation is associated with the synaptic dysfunction observed in depression (Duman and Aghajanian, Reference Duman and Aghajanian2012, Domenici et al., Reference Domenici, Ferrante, Martire, Chiodi, Pepponi, Tebano and Popoli2019), suggesting that DNAm changes differ between brain regions.
In the prefrontal cortex, early maltreatment such as maternal separation increases DNMT activity, DNMT (1, 3a, and 3b) mRNA, and DNMT3a protein levels. These effects were correlated with the DNAm of the BDNF gene (exons IV and IX) and reduced BDNF mRNA in rats (Roth et al., Reference Roth, Lubin, Funk and Sweatt2009, Urb et al., Reference Urb, Anier, Matsalu, Aonurm-Helm, Tasa, Koppel, Zharkovsky, Timmusk and Kalda2019). This stressor also increased global DNAm in the dorsal hippocampus of adolescent male rats and DNAm of BDNF exon IV in the amygdala and ventral hippocampus of female rats (Doherty et al., Reference Doherty, Forster and Roth2016), suggesting sex-specific DNAm changes. In the medial prefrontal cortex of adolescent rats exposed to maltreatment in infancy, BDNF DNAm also increased in males (exon I) (Blaze et al., Reference Blaze, Scheuing and Roth2013).
In addition to the long-lasting effects induced in early development, stressful events in adult animals rapidly modulate DNAm. Footshocks stress increases global DNAm and DNMTs expression in the dorsal hippocampus and prefrontal cortex of rats subjected to the learned helplessness model. These molecular changes in the prefrontal cortex, but not in the dorsal hippocampus, were attenuated by chronic antidepressant treatment (Sales and Joca, Reference Sales and Joca2018). Roth and coworkers (2011) found that predator exposure increases the DNAm of the BDNF promoter in the dorsal hippocampus (dentate gyrus and corn Ammonis, CA1, subregions) and reduces transcript levels of BDNF in the CA1 (Roth et al., Reference Roth, Zoladz, Sweatt and Diamond2011). Acute stress of forced swim also increased DNAm in NR3C1 gene and reduced GR mRNA levels in the hippocampus (dentate gyrus) (Mifsud et al., Reference Mifsud, Saunderson, Spiers, Carter, Trollope, Mill and Reul2017).
Contradictory results including DNA hypomethylation induced by stress and direct correlation between DNAm and gene expression have been found in many studies (Table 2), suggesting that DNAm changes are dependent on multiple factors including stressor, sex, age, brain structure, gene, region, and site methylated. For example, maternal separation stress reduced global DNAm in the nucleus accumbens (Anier et al., Reference Anier, Malinovskaja, Pruus, Aonurm-Helm, Zharkovsky and Kalda2014) and increased DNAm in NR3C1 and Syn I genes following by increased NR3C1 mRNA in hypothalamic neurons (Bockmuhl et al., Reference Bockmuhl, Patchev, Madejska, Hoffmann, Sousa, Sousa, Holsboer, Almeida and Spengler2015), Syn I mRNA, and protein levels in the amygdala (Park et al., Reference Park, Kim, Kang, Chung and Kim2014). Female mice submitted to the maternal exposure to predator odour show decreased DNAm in BDNF exon IV in hippocampus accompanied by elevated CRHR1 in the amygdala and reduced mRNA BDNF in the hippocampus (St-Cyr and McGowan, Reference St-Cyr and Mcgowan2015). In the medial prefrontal cortex of adolescent rats exposed to maltreatment in infancy decreased BDNF DNAm was found in females (exon IV) (Blaze et al., Reference Blaze, Scheuing and Roth2013) while social defeat stress during early adolescence downregulated BDNF expression without altering DNAm of the BDNF IV promoter in adulthood (Xu et al., Reference Xu, Wang, Zhang, Zhao, Ellenbroek, Shao and Wang2018). In adult rats, DNAm is reduced in both males and females (BDNF exon I) and increased in females (BDNF exon IV) submitted to the maternal maltreatment (Blaze et al., Reference Blaze, Scheuing and Roth2013). None BDNF mRNA expression change was observed (Blaze et al., Reference Blaze, Scheuing and Roth2013) suggesting that DNAm may not directly regulate gene transcription leading to an unclear understanding of its functional relevance.
DNAm, such as other epigenetic modifications, has been proposed as a form of genomic metaplasticity preparing the transcriptional response and subsequent neuronal reactivation (reviewed in (Baker-Andresen et al., Reference Baker-Andresen, Ratnu and Bredy2013). Several studies support that DNAm mediates genomic metaplasticity in several ways including the regulation of alternative splicing among others (Oberdoerffer, Reference Oberdoerffer2012). Additionally, in early life, DNMT3a transiently binds across the genome catalysing the DNAm following by binding of methyl-DNA-binding protein MeCP2 in the mice brain. The DNAm within transcribed regions of genes is negatively regulated by gene transcription, and it occurs in a neuronal type-specific manner (Stroud et al., Reference Stroud, Su, Hrvatin, Greben, Renthal, Boxer, Nagy, Hochbaum, Kinde, Gabel and Greenberg2017). The density of DNAm in cytosine-adenine sequences across the genome increases in two specific neuronal subtypes, parvalbumin and vasoactive intestinal peptide expressing interneurons, and it is associated with reduced gene transcription in both neuronal subtypes. However, the increased gene transcription in vasoactive intestinal neuronal peptide and reduced in parvalbumin neurons result in lasting high methylated CA sequences within its transcribed regions in parvalbumin but not vasoactive intestinal peptide neurons (Stroud et al., Reference Stroud, Su, Hrvatin, Greben, Renthal, Boxer, Nagy, Hochbaum, Kinde, Gabel and Greenberg2017) suggesting the indirect gene transcription and DNAm interaction.
Acute stress of forced swim reduced DNAm at CpGs of the c-Fos gene in the hippocampus (Saunderson et al., Reference Saunderson, Spiers, Mifsud, Gutierrez-Mecinas, Trollope, Shaikh, Mill and Reul2016). Roth and coworkers (2011) showed that predator exposure decreases DNAm of the BDNF promoter (CA3) and its mRNA (CA1) in the ventral hippocampus (CA3 subregion) (Roth et al., Reference Roth, Zoladz, Sweatt and Diamond2011). Still, the global hypomethylation and reduced expression of BDNF, GR, and MR are induced by repeated restrain stress in the rat hippocampus (Makhathini et al., Reference Makhathini, Abboussi, Stein, Mabandla and Daniels2017). Chronic stress also results in DNA hypomethylation, reduced DNMT1 RNAm, and protein levels in the lateral habenulae of rats (Shen et al., Reference Shen, Yuan, Wang, Xue, Liu and Zhang2019). In rat prefrontal cortex, chronic unpredictable stress during 2 weeks results in reduced DNMT3a levels, while the overexpression of DNMT3a in stressed rats attenuated impaired stress-induced behaviour and improved the glutamatergic responses (Wei et al., Reference Wei, Cheng, Waddell, Wang, Pang, Cao, Liu, Chitaman, Abreu, Jasrotia, Duffney, Zhang, Dietz, Feng and Yan2020). A significant increase in DNAm of the promoter region of the serotonin 1A receptor (5-HT1A), a regulator of the brain serotonergic tone related to depression, associated with an increase in 5-HT1A mRNA and protein levels are observed in the prefrontal cortex of chronically stressed mice (Le Francois et al., Reference Le Francois, Soo, Millar, Daigle, Le Guisquet, Leman, Minier, Belzung and Albert2015).
Stress also modulates DNAm and gene expression of glutathione peroxidase 1 (Gpx1) and superoxide dismutase1 and 2 (SOD1 and SOD2). The activity of these enzymes is reduced in depressed patients (Herken et al., Reference Herken, Gurel, Selek, Armutcu, Ozen, Bulut, Kap, Yumru, Savas and Akyol2007, Maes et al., Reference Maes, Mihaylova, Kubera, Uytterhoeven, Vrydags and Bosmans2011, Stefanescu and Ciobica, Reference Stefanescu and Ciobica2012, Rybka et al., Reference Rybka, Kedziora-Kornatowska, Banas-Lezanska, Majsterek, Carvalho, Cattaneo, Anacker and Kedziora2013). Chronic mild stress increased the methylation of the Gpx1 promoter and reduced its expression in the blood, whereas DNAm in the SOD1 and SOD2 promoters increased in the hippocampus. The mRNA expression of these genes increased in the brain (hippocampus, amygdala, hypothalamus, midbrain, cortex, basal ganglia (Wigner et al., Reference Wigner, Synowiec, Czarny, Bijak, Jozwiak, Szemraj, Gruca, Papp and Sliwinski2020). Increased mRNA and methylation of the promoter P11, a member of the S100 EF-hand family (Rescher and Gerke, Reference Rescher and Gerke2008), considered a key neuronal modulator in depression and antidepressant response, were also observed in the prefrontal cortex of rats subjected to chronic stress and that are electroconvulsive stimulation (ECS) responsive (Neyazi et al., Reference Neyazi, Theilmann, Brandt, Rantamaki, Matsui, Rhein, Kornhuber, Bajbouj, Sperling, Bleich, Frieling and Loscher2018).
Oxytocin (OXT) and its receptor (OXTR) are proposed to play a relevant role in emotional behaviours, depression, and stress (reviewed in Jurek and Neumann, Reference Jurek and Neumann2018). OXTR gene promoter DNAm significantly decreased in the blood, but not in the brain (hippocampus, striatum, and hypothalamus), of rats reared with low licking compared to those exposed to high licking-grooming (Beery et al., Reference Beery, Mcewen, Macisaac, Francis and Kobor2016) suggesting that the tissue studied can modify the modulation of DNAm complicating its possible role biomarker in depressed individuals.
Clinical studies: DNAm as a possible biomarker for depression
Similarly to stress, depression, and antidepressant drugs have been associated with DNAm alterations (Tables 3 and 4). Genome-wide DNAm association studies are important tools for the identification of genetic associations with complex disorders including MDD, expanding the repertoire of genes, and the alterations related to epigenetic or genetic factors. So, genome-wide association promises significant progress in the understanding of MDD contributing to the identification of biological markers for MDD and response to antidepressant treatments and possible new therapeutic targets (Spreafico et al., Reference Spreafico, Soriaga, Grosse, Virgin and Telenti2020, Uffelmann and Posthuma, Reference Uffelmann and Posthuma2021). The first genome-wide of MDD was reported by Reference Sullivan, De Geus, Willemsen, James, Smit, Zandbelt, Arolt, Baune, Blackwood, Cichon, Coventry, Domschke, Farmer, Fava, Gordon, He, Heath, Heutink, Holsboer, Hoogendijk, Hottenga, Hu, Kohli, Lin, Lucae, Macintyre, Maier, Mcghee, Mcguffin, Montgomery, Muir, Nolen, Nothen, Perlis, Pirlo, Posthuma, Rietschel, Rizzu, Schosser, Smit, Smoller, Tzeng, Van Dyck, Verhage, Zitman, Martin, Wray, Boomsma and PenninxSullivan et al. (2009), and the first genome-wide DNAm scan in MDD, covering 3.5 million CpGs, was published in 2012 (Sabunciyan et al., Reference Sabunciyan, Aryee, Irizarry, Rongione, Webster, Kaufman, Murakami, Lessard, Yolken, Feinberg, Potash and Gen2012). Since then, an increasing number of studies about MDD and antidepressant response have identified DNAm differences in candidate regions (differentially methylated sites) for several genes (Numata et al., Reference Numata, Ishii, Tajima, Iga, Kinoshita, Watanabe, Umehara, Fuchikami, Okada, Boku, Hishimoto, Shimodera, Imoto, Morinobu and Ohmori2015), including neuronal development genes (Sabunciyan et al., Reference Sabunciyan, Aryee, Irizarry, Rongione, Webster, Kaufman, Murakami, Lessard, Yolken, Feinberg, Potash and Gen2012, Weder et al., Reference Weder, Zhang, Jensen, Yang, Simen, Jackowski, Lipschitz, Douglas-Palumberi, Ge, Perepletchikova, O’loughlin, Hudziak, Gelernter and Kaufman2014) and plasticity (Weder et al., Reference Weder, Zhang, Jensen, Yang, Simen, Jackowski, Lipschitz, Douglas-Palumberi, Ge, Perepletchikova, O’loughlin, Hudziak, Gelernter and Kaufman2014), genes that encode cell adhesion molecules and neurotransmitter receptors (Oh et al., Reference Oh, Chambwe, Klein, Gal, Andrews, Gleason, Shaknovich, Melnick, Campagne and Toth2013), immune response-related genes (Nemoda et al., Reference Nemoda, Massart, Suderman, Hallett, Li, Coote, Cody, Sun, Soares, Turecki, Steiner and Szyf2015), and others (Yang et al., Reference Yang, Zhang, Ge, Weder, Douglas-Palumberi, Perepletchikova, Gelernter and Kaufman2013, Davies et al., Reference Davies, Krause, Bell, Gao, Ward, Wu, Lu, Liu, Tsai, Collier, Murphy, Dempster, Mill, Consortium, Battle, Mostafavi, Zhu, Henders, Byrne, Wray, Martin, Spector and Wang2014, Dempster et al., Reference Dempster, Wong, Lester, Burrage, Gregory, Mill and Eley2014, Cordova-Palomera et al., Reference Cordova-Palomera, Fatjo-Vilas, Gasto, Navarro, Krebs and Fananas2015, Ju et al., Reference Ju, Fiori, Belzeaux, Theroux, Chen, Aouabed, Blier, Farzan, Frey, Giacobbe, Lam, Leri, Macqueen, Milev, Muller, Parikh, Rotzinger, Soares, Uher, Li, Foster, Kennedy and Turecki2019). Although additional studies are needed, genome-wide analysis allows for the changes in specific genes, identifying the genomic and transcriptomic profiles for MDD, and thus contributing to the further understanding of pathways associated with complex psychiatric illnesses including depression. In this context, epigenetic studies in animals and humans suggest that the DNAm of specific genes could be a potential marker of several disorders, including depression. Integrative DNA methylome and transcriptome analysis found 39 differentially methylated regions (DMRs) and 30 differentially expressed genes (DEGs) in genes associated with signalling pathways related to stress responses, neuron apoptosis, the insulin receptor, mTOR, and nerve growth factor receptor signalling, in peripheral blood monocytes of monozygotic twin pairs with a lifetime history of MDD. These findings were replicated in the postmortem brain (dorsal lateral prefrontal cortex, BA9) of suicide individuals with MDD (Zhu et al., Reference Zhu, Strachan, Fowler, Bacus, Roy-Byrne and Zhao2019). Additionally, the genome-wide analysis revealed 366 DMRs of genes associated with learning, memory, and behaviour in the hippocampus of suicide individuals (Labonte et al., Reference Labonte, Suderman, Maussion, Lopez, Navarro-Sanchez, Yerko, Mechawar, Szyf, Meaney and Turecki2013), suggesting the DNAm association and its potential biomarker role for depression.
Table 3. Studies showing modulation and increased DNA methylation (DNAm) in depression

Note: MDD, major depressive disorder; DNAm, DNA methylation; CpG, cytosine-phosphate-guanine; mRNA, messenger RNA.
Table 4. Studies showing no change, decreased DNA methylation (DNAm), and complex gene expression modulation by DNAm in depression

Note: DNAm, DNA methylation; MDD, major depressive disorder; CpG, cytosine-phosphate-guanine.
Prenatal bisphenol A exposure results in disturbed emotional regulation, aggressive behaviour, and induces long-lasting BDNF DNAm alterations in the blood and brain (hippocampus) of mice. Still, increased DNAm in two CpG sites of BDNF IV was observed in the cord blood of humans exposed to high maternal bisphenol A levels in utero (Perera et al., Reference Perera, Vishnevetsky, Herbstman, Calafat, Xiong, Rauh and Wang2012, Kundakovic et al., Reference Kundakovic, Gudsnuk, Herbstman, Tang, Perera and Champagne2015). Mothers with persistent perinatal depression, but not their children, have increased OXTR DNAm in the saliva (King et al., Reference King, Robins, Chen, Yerko, Zhou, Nagy, Feeley, Gold, Hayton, Turecki and Zelkowitz2017). However, individuals exposed to prenatal or early stress (child maltreatment) presented increased DNAm in four CpG sites of the serotonin transporter gene-linked polymorphic region (5-HTTLPR) promoter. This change was associated with decreased 5-HTTLPR mRNA levels in the peripheral blood cells (Wankerl et al., Reference Wankerl, Miller, Kirschbaum, Hennig, Stalder and Alexander2014).
A higher DNAm in the BDNF promoter region was associated with suicidal ideation and previous suicidal attempt history in MDD patients (Kang et al., Reference Kang, Kim, Lee, Kim, Bae, Kim, Shin, Kim, Shin and Yoon2013a). In the BDNF IV promoter, increased DNAm in the postmortem brain of suicide individuals was related to lower BDNF mRNA levels in the Wernicke area (Keller et al., Reference Keller, Sarchiapone, Zarrilli, Videtic, Ferraro, Carli, Sacchetti, Lembo, Angiolillo, Jovanovic, Pisanti, Tomaiuolo, Monticelli, Balazic, Roy, Marusic, Cocozza, Fusco, Bruni, Castaldo and Chiariotti2010). MDD suicides also shown DNA hypermethylation in the cortex. The hypermethylation of TrkB promoter is associated with a lower TrkB expression in the frontal cortex (Ernst et al., Reference Ernst, Deleva, Deng, Sequeira, Pomarenski, Klempan, Ernst, Quirion, Gratton, Szyf and Turecki2009), and the increased DNAm in the prefrontal cortex (Brodmann Area 47, BA47) is positively correlated with age suggesting that DNAm alterations are age dependent (Haghighi et al., Reference Haghighi, Xin, Chanrion, O’donnell, Ge, Dwork, Arango and Mann2014).
Contradictory results in the modulation of DNAm in MDD have been shown in many studies (Table 4). Mothers with persistent perinatal depression have hypomethylation in intergenic regions of the arginine vasopressin (AVP) gene, a neuropeptide involved in maternal behaviour and stress regulation, in the saliva (Bachner-Melman and Ebstein, Reference Bachner-Melman and Ebstein2014, Bridges, Reference Bridges2015, King et al., Reference King, Robins, Chen, Yerko, Zhou, Nagy, Feeley, Gold, Hayton, Turecki and Zelkowitz2017). None significant genome-wide association was observed between maternal depressive symptoms and infant DNAm (Wikenius et al., Reference Wikenius, Myhre, Page, Moe, Smith, Heiervang, Undlien and Leblanc2019). However, infants of mothers with MDD showed decreased BDNF IV DNAm (Braithwaite et al., Reference Braithwaite, Kundakovic, Ramchandani, Murphy and Champagne2015).
DNAm alterations also were found in peripheral tissues of MDD patients. Glucocorticoid receptor gene (NR3C1 exon 1F) was hypermethylated in the blood of MDD patients (Farrell et al., Reference Farrell, Doolin, Jairaj, Roddy, Tozzi, Morris, Harkin, Frodl, Nemoda, Szyf, Booij and O’keane2018). NR3C1 promoter hypermethylation was also observed in the blood of females, but not in males, MDD patients (Nantharat et al., Reference Nantharat, Wanitchanon, Amesbutr, Tammachote and Praphanphoj2015), indicating a gender influence.
Genome-wide analysis shown reduced DNAm in 365 CpG sites in the blood of MDD patients (Numata et al., Reference Numata, Ishii, Tajima, Iga, Kinoshita, Watanabe, Umehara, Fuchikami, Okada, Boku, Hishimoto, Shimodera, Imoto, Morinobu and Ohmori2015). DNA hypomethylated was observed in specific genes including NR3C1 and BDNF of patients with MDD. NR3C1 promoter was hypomethylated at two specific CpG sites in the peripheral blood of these patients (Na et al., Reference Na, Chang, Won, Han, Choi, Tae, Yoon, Kim, Joe, Jung, Lee and Ham2014), and the BDNF hypomethylation was found in the complete gene (Song et al., Reference Song, Miyaki, Suzuki, Sasaki, Tsutsumi, Kawakami, Shimazu, Takahashi, Inoue, Kan, Kurioka and Shimbo2014) and exon I promoter in the peripheral blood (Fuchikami et al., Reference Fuchikami, Morinobu, Segawa, Okamoto, Yamawaki, Ozaki, Inoue, Kusumi, Koyama, Tsuchiyama and Terao2011) and saliva of persons with more severe MDD compared with a less severe disorder (Song et al., Reference Song, Miyaki, Suzuki, Sasaki, Tsutsumi, Kawakami, Shimazu, Takahashi, Inoue, Kan, Kurioka and Shimbo2014). However, no correlation was observed between BDNF promoter methylation and its levels in the serum of MDD patients (Na et al., Reference Na, Won, Kang, Chang, Yoon, Tae, Kim, Lee, Joe, Kim and Ham2016). Additionally, Cruceanu and colleagues (Reference Cruceanu, Kutsarova, Chen, Checknita, Nagy, Lopez, Alda, Rouleau and Turecki2016) found significant DNA hypomethylation in the prefrontal cortex (BA10) of suicidal individuals with bipolar disorder or MDD compared with psychiatrically healthy individuals. Furthermore, the study showed an inverse correlation between the DNAm of the SYN2 gene and its mRNA expression (Cruceanu et al., Reference Cruceanu, Kutsarova, Chen, Checknita, Nagy, Lopez, Alda, Rouleau and Turecki2016).
Candidate gene selection related to stress and depression based on prior knowledge is often used; however, genome-wide studies have reported controversial findings and a large number of associations in other genes (Sullivan et al., Reference Sullivan, Eaves, Kendler and Neale2001, Sullivan, Reference Sullivan2007, Sullivan, Reference Sullivan2017, Border et al., Reference Border, Johnson, Evans, Smolen, Berley, Sullivan and Keller2019) including genes encoding lysophosphatidic acid receptor (LPAR2), related with diverse cellular activities (Fukushima et al., Reference Fukushima, Kado, Tsujiuchi and Choi2018), and adaptor-associated Kinase 1 (AAK1), associated with the intracellular trafficking of multiple viruses (Verdonck et al., Reference Verdonck, Pu, Sorrell, Elkins, Froeyen, Gao, Prugar, Dorosky, Brannan, Barouch-Bentov, Knapp, Dye, Herdewijn, Einav and De Jonghe2019, Zhu et al., Reference Zhu, Strachan, Fowler, Bacus, Roy-Byrne and Zhao2019). Additional studies are needed since the methylation rate of these genes may identify novel targets for antidepressant drugs, diverse signalling in stress-related pathologies, and display predictive function for evaluating the antidepressant response.
Effects of antidepressant drugs on DNAm
DNAm profile has been proposed as a factor influencing both the neurobiology of depression and antidepressant treatment response. In fact, DNAm changes at specific CpG sites have been documented for antidepressant drugs. Although few studies have investigated the potential of DNAm as a biomarker of treatment response, data support this hypothesis for antidepressants (Table 5).
Table 5. Antidepressant´s effects on DNAm

Note: qPCR, quantitative polymerase chain reaction; MDD, major depressive disorder; DNAm, DNA methylation; CpG, cytosine-phosphate-guanine; DNMT, DNA methyltransferase enzyme; ELISA, Enzyme-Linked Immunosorbent Assay; MEDIP, methylated DNA immunopreciptation; mRNA, messenger RNA.
Table 6 Effects of DNAm inhibition in the stress and depression.

Note: ELISA, enzyme-linked immunosorbent assay; DNAm, DNA methylation; AMPA, α-amino-3-hydroxy-5-methyl-4-isoxazolepropionic acid receptor; DNMT, DNA methyltransferase enzyme; MEDIP, methylated DNA immunoprecipitation; qPCR, quantitative polymerase chain reaction.
Preclinical studies
Supporting the involvement of DNAm in the action of antidepressant, in vitro cortical astrocytes treated with antidepressant drugs shown reduced DNMT1 activity (Zimmermann et al., Reference Zimmermann, Zschocke, Perisic, Yu, Holsboer and Rein2012). Other studies have shown that antidepressant drugs attenuate stress-induced hypermethylation in animals. For instance, results from an in vivo study identified that perinatal exposure to paroxetine, a selective serotonin reuptake inhibitor, leads to DNA hypomethylation in several genes including plasticity-related genes, and reduces DNMT3a mRNA in the hippocampus during the early of rats life (Glover et al., Reference Glover, Mccoy, Shupe, Unroe, Jackson and Clinton2019). In Flinders Sensitive Line rats, a genetic model of depression, Melas and coworkers (Reference Melas, Rogdaki, Lennartsson, Bjork, Qi, Witasp, Werme, Wegener, Mathe, Svenningsson and Lavebratt2012) shown increased DNAm in the P11 promoter and decreased P11 levels in the prefrontal cortex, both reversed by chronic escitalopram treatment (Melas et al., Reference Melas, Rogdaki, Lennartsson, Bjork, Qi, Witasp, Werme, Wegener, Mathe, Svenningsson and Lavebratt2012). Still, antidepressant drugs (fluoxetine, desipramine, and imipramine) attenuated stress-induced changes in DNAm levels in the hippocampus and prefrontal cortex of rodents (Sales and Joca, Reference Sales and Joca2016, Sales and Joca, Reference Sales and Joca2018). Footshocks stress increased total DNAm and DNMTs (DNMT 3a and DNMT 3b) levels in the dorsal hippocampus and prefrontal cortex while repeated imipramine treatment attenuated their changes only in the prefrontal cortex (Sales and Joca, Reference Sales and Joca2018). In the prefrontal cortex of chronically stressed mice, the stress-induced 5-HT1A DNA hypermethylation and reduced 5-HT1A expression were reversed by chronic imipramine treatment (Le Francois et al., Reference Le Francois, Soo, Millar, Daigle, Le Guisquet, Leman, Minier, Belzung and Albert2015). Chronic despair mouse model reduced mRNA and increased promoter DNAm of Homer1, a synaptic plasticity protein related with depression and action of antidepressant drugs (Serchov et al., Reference Serchov, Clement, Schwarz, Iasevoli, Tosh, Idzko, Jacobson, De Bartolomeis, Normann, Biber and Van Calker2015, Serchov et al., Reference Serchov, Heumann, Van Calker and Biber2016) in the cortex and blood (Sun et al., Reference Sun, Verkaik-Schakel, Biber, Plosch and Serchov2020). However, chronic antidepressant treatment reduced Homer1 promoter DNAm and expression in the cortex (Sun et al., Reference Sun, Verkaik-Schakel, Biber, Plosch and Serchov2020), suggesting that the DNAm is brain region-specific and that the regulation of DNAm in the cortex is an important mechanism associated with the antidepressant effect.
Contradictory results demonstrate that antidepressant drugs can increased DNAm. Rat offspring exposed to fluoxetine during pregnancy and lactation showed decreased global DNAm in the hippocampus and increased DNAm in the cortex (Toffoli et al., Reference Toffoli, RodriguesOliveira, JrOliveira, Silva, Moreira, Pelosi and Gomes2014), suggesting long-lasting and region-specific DNAm changes induced by antidepressants. Contrary to this, the exposure to fluoxetine during gestation and lactation increased global DNAm in the hippocampus, reduced social interaction time, and decreased plasma corticosterone levels of male offspring subjected to the restraint stress (Silva et al., Reference Silva, Toffoli, Estrada, Verissimo, Francis-Oliveira, Moreira, Gomes and Pelosi2018). Still, increased BDNF expression is associated with increased DNMT1 activity in blood cells of MDD patients treated ex vivo with paroxetine (Gassen et al., Reference Gassen, Fries, Zannas, Hartmann, Zschocke, Hafner, Carrillo-Roa, Steinbacher, Preissinger, Hoeijmakers, Knop, Weber, Kloiber, Lucae, Chrousos, Carell, Ising, Binder, Schmidt, Ruegg and Rein2015).
Clinical studies
No global DNAm difference was observed between MDD patients and healthy controls or between medicated and unmedicated individuals with MDD (Okada et al., Reference Okada, Morinobu, Fuchikami, Segawa, Yokomaku, Kataoka, Okamoto, Yamawaki, Inoue, Kusumi, Koyama, Tsuchiyama, Terao, Kokubo and Mimura2014). However, genome-wide analyses revealed differentially methylated sites and expressed genes in the blood of MDD escitalopram responders (Ju et al., Reference Ju, Fiori, Belzeaux, Theroux, Chen, Aouabed, Blier, Farzan, Frey, Giacobbe, Lam, Leri, Macqueen, Milev, Muller, Parikh, Rotzinger, Soares, Uher, Li, Foster, Kennedy and Turecki2019). MDD individuals who best respond to the selective serotonin reuptake inhibitor, paroxetine, showed 623 CpG sites differently methylated (Takeuchi et al., Reference Takeuchi, Nonen, Kato, Wakeno, Takekita, Kinoshita and Kugawa2017). Individual variations in the antidepressant responses were related to these DNAm changes in the blood (Takeuchi et al., Reference Takeuchi, Nonen, Kato, Wakeno, Takekita, Kinoshita and Kugawa2017), suggesting that DNAm alterations at specific genes could be associated with antidepressant drugs being predictive and biomarker of therapeutic response.
Generally, in humans, the MDD-related hypermethylation is not attenuated by antidepressant treatment and curiously the antidepressant response can be associated with increased DNAm in specific genes. Hsieh and coworkers (Reference Hsieh, Lin, Lee and Huang2019) found that MDD is associated with lower serum BDNF mRNA and protein levels in the blood (Hsieh et al., Reference Hsieh, Lin, Lee and Huang2019). In this study, patients with MDD showed different DNAm between the CpG sites of BDNF exon IX promoter (Hsieh et al., Reference Hsieh, Lin, Lee and Huang2019). BDNF promoter DNA hypermethylation was reported in the blood of patients with MDD (Carlberg et al., Reference Carlberg, Scheibelreiter, Hassler, Schloegelhofer, Schmoeger, Ludwig, Kasper, Aschauer, Egger and Schosser2014), while antidepressant therapy further increased DNAm in MDD individuals compared with MDD individuals without antidepressant treatment (Carlberg et al., Reference Carlberg, Scheibelreiter, Hassler, Schloegelhofer, Schmoeger, Ludwig, Kasper, Aschauer, Egger and Schosser2014). In fact, reduced BDNF DNAm is associated with impaired response of antidepressant escitalopram (Wang et al., Reference Wang, Zhang, Lv, Bao, Sun, Ma, Fang, Yi and Cai2018b), while the better antidepressant responses are related to DNA hypermethylation in two CpG sites of BDNF IX suggesting a site-dependent DNAm (Hsieh et al., Reference Hsieh, Lin, Lee and Huang2019). In BDNF IV, the resistance to antidepressant response is related to reduced DNAm (CpG87) and lower BDNF expression in the blood of MDD patients (Domschke et al., Reference Domschke, Tidow, Schwarte, Deckert, Lesch, Arolt, Zwanzger and Baune2014, Tadic et al., Reference Tadic, Muller-Engling, Schlicht, Kotsiari, Dreimuller, Kleimann, Bleich, Lieb and Frieling2014). It is not clear, however, how these results can explain the well demonstrated association of low BDNF levels with depression (Lin and Huang, Reference Lin and Huang2020, Notaras and van den Buuse, 2020).
The serotonin transporter gene (SLC6A4), responsible for serotonin reuptake, has been related to interindividual differences in antidepressant treatment responses (Taylor et al., Reference Taylor, Sen and Bhagwagar2010, Tolsma and Hansen, Reference Tolsma and Hansen2019). DNA hypermethylation at the SLC6A4 (promoter and CpG 3) found in patients with MDD before six weeks, but not twelve weeks (Kang et al., Reference Kang, Kim, Stewart, Kim, Bae, Kim, Shin, Shin and Yoon2013b), of antidepressant treatment, was related with better therapeutic response possibility increasing serotonin levels in the synaptic cleft (Domschke et al., Reference Domschke, Tidow, Schwarte, Deckert, Lesch, Arolt, Zwanzger and Baune2014, Okada et al., Reference Okada, Morinobu, Fuchikami, Segawa, Yokomaku, Kataoka, Okamoto, Yamawaki, Inoue, Kusumi, Koyama, Tsuchiyama, Terao, Kokubo and Mimura2014, Schiele et al., Reference Schiele, Zwanzger, Schwarte, Arolt, Baune and Domschke2020). These results suggest that DNAm levels in specific sites of SLC6A4 can be associated with antidepressant response during a specific time of treatment. Additionally, impaired escitalopram response is related to 5-HT1A and 5-HT1B DNA hypomethylation of two CpG sites (5-HT1a CpG 668 and 5-HTR1b CpG 1401) in the blood of MDD patients possibility increasing their expression and reducing 5-HT levels which might counteract the antidepressant effects of escitalopram (Wang et al., Reference Wang, Lv, Mao, Zhang, Bao, Sun, Chen, Yi, Cai and Fang2018a).
Together, these data indicate that DNAm can be influenced by antidepressant drugs in MDD patients, and this can be associated with its therapeutic response. However, more studies are needed in order to understand the predictive value of DNAm changes in depression and its potential use as a biomarker in antidepressant responses.
Effects of the pharmacological manipulation of DNAm in stress and depression
Supporting the involvement of DNAm in the stress responses, inhibition of DNAm with central or peripheral injections of DNMTi results in antidepressant-like effects. The acute systemic administration of two different and chemically unrelated DNMTi (5-AzaD and RG108) promoted stress-coping behaviour in mice exposed to the forced swim test and tail suspension test, smililary to other antidepressants (Sales et al., Reference Sales, Biojone, Terceti, Guimaraes, Gomes and Joca2011, Sales and Joca, Reference Sales and Joca2016). These effects were associated with increased BDNF levels in rat hippocampus (Sales et al., Reference Sales, Biojone, Terceti, Guimaraes, Gomes and Joca2011). In another study, single injection of 5-AzaD and RG108 induces rapid (1h) and long-lasting (7 days after) antidepressant-like effects in rats exposed to the learned helplessness model (Sales et al., Reference Sales, Maciel, Suavinha and Joca2020). Interestingly, the RG108 treatment attenuated both increased DNAm and reduced BDNF IV and TrkB expression induced by stress in the prefrontal cortex. Moreover, the behavioural effects induced by RG108 were blocked by TrkB antagonism or mTOR inhibition in the medial prefrontal cortex (Sales et al., Reference Sales, Maciel, Suavinha and Joca2020), suggesting that the fast disinhibition of BDNF-TrkB-mTOR signalling can be associated with antidepressant-like effects of DNMTi. DNMTi (5-AzaD and RG108) microinjected intracerebroventricularly (Dong et al., Reference Dong, Locci, Gatta, Grayson and Guidotti2019) or directly into the hippocampus (Sales et al., Reference Sales, Biojone, Terceti, Guimaraes, Gomes and Joca2011) and nucleus accumbens (LaPlant et al., Reference Laplant, Vialou, Covington, Dumitriu, Feng, Warren, Maze, Dietz, Watts, Iniguez, Koo, Mouzon, Renthal, Hollis, Wang, Noonan, Ren, Eisch, Bolanos, Kabbaj, Xiao, Neve, Hurd, Oosting, Fan, Morrison and Nestler2010) also induced antidepressant-like effects, thus indicating that the drugs themselves are promoting the antidepressant effect rather than some product of their metabolism.
Contrary to this, local 5-AzaD infusion into the ventrolateral orbital cortex (Xing et al., Reference Xing, Liu, Xu, Xu and Dang2014) and lateral habenulae (Shen et al., Reference Shen, Yuan, Wang, Xue, Liu and Zhang2019) resulted in depressive-like behaviours and decreased global DNAm. DNMT1 mRNA expression and protein levels also were reduced in the lateral habenulae (Shen et al., Reference Shen, Yuan, Wang, Xue, Liu and Zhang2019). Still, the DNMTi-induced hypomethylation in the lateral habenulae is followed by reduced 5-HT and 5-HIAA levels in the dorsal raphe nucleus (Shen et al., Reference Shen, Yuan, Wang, Xue, Liu and Zhang2019). Furthermore, unstressed rats treated with DNMTi shown similar effects to the chronically stressed rats including reduced AMPA receptor expression in the prefrontal cortex (Wei et al., Reference Wei, Cheng, Waddell, Wang, Pang, Cao, Liu, Chitaman, Abreu, Jasrotia, Duffney, Zhang, Dietz, Feng and Yan2020).
Such differences can reflect the differential expression of DNMT subtypes in the brain associated with the lack of selectivity of the drugs for the different DNMTs (Xu et al., Reference Xu, Hu, Luo and Liang2016, Zhou et al., Reference Zhou, Li and Liu2018). This is supported, for example, but studies in which conditional deletion of DNMT1 or DNMT3 produced different behavioural results, as shown by Morris and colleagues (Morris et al., Reference Morris, Na, Autry and Monteggia2016). In this study, conditional forebrain knockout DNMT1 mice presented anxiolytic and antidepressant-like behaviour in different animal models, whereas DNMT3a knockout mice did not show any significant behavioural changes in the same tests. This study highlights the importance of developing selective drugs that could allow the investigation of the differential participation of DNMTs subtypes as possible therapeutic targets in depression. Another possibility would be to use epigenome editing tools to selectively target the different DNMTs and therefore regulate DNAm in a more specific way. In a recent work developed by Lin et al. (Reference Lin, Liu, Xu, Huang, Daugaard, Petersen, Hansen, Ye, Zhou, Fang, Yang, Li, Floe, Jensen, Shrock, Chen, Yang, Wang, Liu, Xu, Bolund, Nielsen and Luo2018), DNMT domains were fushioned to the nuclease-deficient clustered regularly interspaced short palindromic repeat (CRISPR) associated protein 9 (Cas9) as a way to regulate DNAm induced only by DNMTa. Similar approaches of gene therapy could represent a new perspective for targeting DNAm in stress-related psychiatric disorders, including depression.
Limitations
The data are consistent in the context of stress, depression, and antidepressant drugs modulate DNAm. However, contradictory results are currently found. It has been suggested that DNAm alterations are influenced by diverse factors, including the developmental stage, the methylated region of gene, tissue, region, cell type, sex, age, and stressor (type, duration, and intensity). To date, the majority of studies evaluate a limited number of regions and sites within a specific gene. Still, generally, studies investigating DNAm in the brain and peripheral blood use whole tissue and brain region, lysates, or only leukocytes without considering cell type-specific DNAm changes. In fact, methylation studies are typically performed in tissue containing multiple cell types. However, the analysis of cell-type specificity on DNAm is relevant for better interpretation of results, the understanding of stress responses and neurobiology of depression, and identifying new antidepressant targets since the neuronal diversity in the brain (Tasic et al., Reference Tasic, Yao, Graybuck, Smith, Nguyen, Bertagnolli, Goldy, Garren, Economo, Viswanathan, Penn, Bakken, Menon, Miller, Fong, Hirokawa, Lathia, Rimorin, Tieu, Larsen, Casper, Barkan, Kroll, Parry, Shapovalova, Hirschstein, Pendergraft, Sullivan, Kim, Szafer, Dee, Groblewski, Wickersham, Cetin, Harris, Levi, Sunkin, Madisen, Daigle, Looger, Bernard, Phillips, Lein, Hawrylycz, Svoboda, Jones, Koch and Zeng2018) and microcircuit cell type specific related to stress and MDD (Tremblay et al., Reference Tremblay, Lee and Rudy2016) have confusing potential in the results of studies. Cell-type-specific methylome-wide association studies identified differences in sub-populations of neurons/glia and granulocytes/T-cells/B-cells/monocytes for the human brain and blood samples (Chan et al., Reference Chan, Turecki, Shabalin, Guintivano, Zhao, Xie, Van Grootheest, Kaminsky, Dean, Penninx, Aberg and Van Den Oord2020). The analyses in the T-cells, monocytes, and glia showed novel MDD-methylation associations in signalling involved in the immune system, nerve growth factor (NGF) and its receptor p75NTR, and innate immune Toll-like receptors (TLR) in both blood and brain of MDD patients (Chan et al., Reference Chan, Turecki, Shabalin, Guintivano, Zhao, Xie, Van Grootheest, Kaminsky, Dean, Penninx, Aberg and Van Den Oord2020). Moreover, the methods for determining the DNAm profile differ across studies. These differential factors could help to explain some of the contradictory results in the literature.
Perspectives and conclusions
DNAm is a promising mechanism for the prediction of biomarkers of depression and antidepressant response. Additionally, DNAm is important for understanding of MDD neurobiology, and its modulation could be used for the development of new pharmacological tools in psychiatry. Although several findings support the relevance of DNAm, many contradictory results are found, possibly because of the high specificity of DNAm confusing the interpretation of the data. Therefore, new studies using genome-wide methylation analysis of specific cell types associated with the multi-omics approach could better point to more relevant changes in DNAm associated with stress and depression suggesting that cell-type specific DNAm analyses are relevant for biological knowing providing mechanistic insights into stress and depression. The biggest challenge has been the identification of causal mechanisms since DNAm changes in specific loci suggest the relevance for depression without direct relation to underlying biological function. Moreover, the use of genome editing tools, such as CRISPR-Cas9 based DNAm modifiers, could reveal the potential of targeting the epigenome in the search for better and more effective antidepressant treatments.
Acknowledgements
This work was supported by grants from FAPESP (2018/15896-3), CAPES, CNPq, and AUFF (AUFF-E-2020-7-19).
Disclosure statement
The authors declare no conflict of interest.
Author contributions
All authors wrote and agreed on the final version of the manuscript.